The term lignocellulose is used to refer the plant's cell wall, which includes hemicellulose 17-32% and cellulose 38-50% which is composed of hexose and pentose sugar monomers and lignin 15-30% which is comprised of aromatic polyphenol [1]. The cell walls are made up of cellulose and hemicellulose, which work together through covalent bonding to cross-link non-cellulosic and cellulosic polymers as shown in Figure 1. Polysaccharide polymers are held together by the "glue" of lignin, which is a gum that mimics formaldehyde resin. This provides the cell walls with extra mechanical strength to withstand against insects or moist conditions. In addition, lignin serves as the cell wall's hydrophobic barrier, allowing water and several vital nutrients to pass through. Polymeric compounds have been found in higher quantities in the plant's central and secondary cell walls, despite the fact that the fractional arrangement might vary substantially depending on the plant's genetics, species, and on environmental variables [2].
Sources and components of lignocellulose
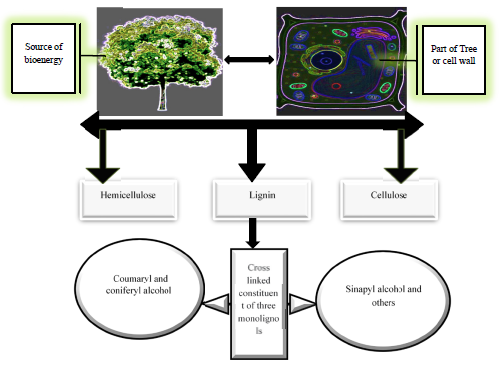
Lignocellulosic bioenergy exploration has grown at an exponential rate in recent years due to the depleting fossil fuel supplies and environmental damage caused by the depletion of these resources. Furthermore, petroleum consumption per day has been growing at considerably rapid pace (Figure 2) due to expanding populations and displacement of human work to machinery. Many renewable energy sources have been studied so far in an effort to progressively shift to a bio-based economy, including hydrothermal. To now, the only viable renewable energy source that has the prospective to exchange petroleum-based fuel in transportation or chemical production is biomass-derived energy.
Global petroleum consumption rate per day on average basis from 2015 to 2022 [3].
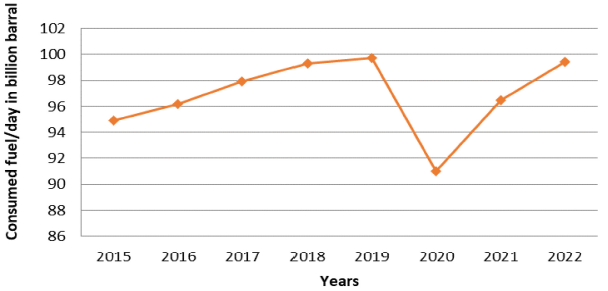
Lignocellulosic biomass has shown to be an effective source of renewable bioenergy [4] due to the high chemical energy it contains [5] and due to the 75 percent carbohydrate content of cellulose and hemicellulose. No doubt, it enhances the bioenergy production by various mechanism and requires extra economic cost and environmental issue. Its environmental issue and economic cost are affordable as compared to other conventional methods and technologies. So that's why, nanobiotechnological trend is increasing due to their less environmental impacts economic effects. The schematic trend in which biomass converts into bioenergy has been shown in Figure 3.
Schematic explanation of the conversion of biomass to bioenergy by nanotechnology
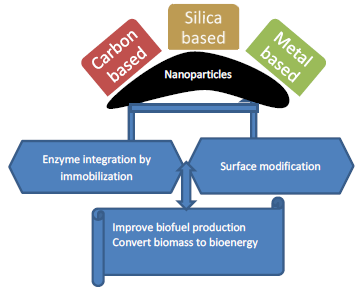
Biorefining also yields furfural, levulinic acid, and 5-hydroxymethylfurfural, all of which are excellent building blocks for the chemical or biological production of energy sources [6]. In contrast, with an estimated annual output of 1.7-2 x 1011 tonnes per year from agriculture and forestry, lignocellulosic biomass is readily accessible [6]. Out of the 1.2 billion metric ton of biomass produced by diverse crops in 2011, the EU utilized 38 million ton for biofuels [7]. It is also estimated that by 2030, the United States alone would have 450 million dry tonnes of lignocellulose biomass, which can produce 67 Ggal of ethanol annually [8]. On the other hand, synthetic biofuel production may result in climate change regime that is one of the hot issues in today's modern world. Its sources may be agriculture, inorganic forming and etc. that are the major causes.
In spite of these benefits, cellulose crystallinity and nonreactive lignin make biomasses intractable in nature. Prior to the biofuel production procedures, cellulose must be pretreated to make it more susceptible to the enzymatic hydrolysis process, which then aids in the extraction of fermentable sugars [9]. High costs and inadequate infrastructure have also hampered efforts to produce bioenergy from lignocellulosic material with a high level of quality and yield. With these restrictions, research has documented numerous optimization techniques for pretreatment and enzymes and fermentation phases in order to increase bioenergy production in energy efficient and cost-effective way." Bioenergy production has benefited greatly from recent advances in nanomaterials and their unique features [9]. As a result, the basic concepts of nanomaterials and their importance in the creation of bioenergy are comprehensively covered in this paper. It has also been stated that these approaches may be combined depending on the procedure. Next, we'll look at how a nanobiocatalyst can be used to generate bioenergy. Finally, the constraints and opportunities for bioenergy production from lignocellulosic biomasses are presented.
In its crystallized state, cellulose is made up of densely packed glucose monomers that are bound together by 1-4 glycosidic bonds to create tight polymer chains. Cellulose is arranged into amorphous regions to some extent by inter molecular hydrogen bonding [10]. The large number of hydroxyl functional group at its lateral fibres encourages the formation of hydrogen bonds, which makes it more stable and, consequently, more resistant to depolymerization. In lignocellulosic biomass, it accounts for 30-50 percent of the total. Hemicellulose is a branching carbohydrate that is amorphous and less polymerized than other polysaccharides found in the plant kingdom. Because of this characteristic, it is more prone to breakdown than cellulose. A five- or six-carbon sugar chain is randomly scattered throughout the structure, with xylan serving as the primary structural unit. Lignocellulosic biomass (LCB) is made up of cellulose fibres that are linked together non-covalently by hemicellulose, which serves as a matrix material in the creation of LCB. Hemicellulose accounts for 20-30 percent of the total volume of LCB [11]. Lignin is the world's second most prevalent hydrophobic polymer, after cellulose [12], and it may be found in a wide range of plant and animal tissues. It is a heteropolymer composed of monomers of alcohols such as p-coumaryl, coniferyl, and sinapyl, among others [13]. It functions as a glue in LCB, binding together cellulose and hemicellulose fibres by filling the gaps between them, resulting in a three-dimensionally cross-linked and extremely stable molecular structure.
As a result of its high stability, it is stubborn and resistant to degradation [14]. In order to estimate the biorefinery potential of LCB, it is necessary to first establish the chemical composition of the biomass. In addition to having a reduced lignin concentration (less than 20% by weight), agricultural leftovers [15] such as sugar cane straw [16], rice straw [17], and wheat straw [18] have been reported to be extensively employed owing to their large amounts of cellulosic, hemicellulose contents and other biobased products. Feedstock, on the other hand, include a high concentration of biofuel production such as bioethanol [19].
Nanomaterials are described as substances whose exterior, interior, or apparent structural dimensions in the nanoscale range regardless of their composition. Nanomaterials characterization based on size, origin, composition and shape [20]. Nanoparticles (NPs) may be generically divided into four types: carbonic, natural or organic, composite and inorganic. Carbonic NPs are those that are made of graphene and its oxides, nano diamonds [21], organic NPs is composed of synthetic or natural molecules, inorganic from elements (metals, non-metals and oxides) and composite from both organic shell and inorganic core (hybrid). The chemical structure of NPs determines their physical features, which in turn impacts their functioning in different situations [22]. Furthermore, NPs have quite different physical and chemical properties [23] than macro particles, which may be applied in a variety of industries such as medicine, bioenergy, ionic liquids, electronics, and consumer goods. Moreover, despite their crucial role in the bioenergy generation process, NPs ensure a quicker response time than larger particles. In part due to their small particle sizes, NPs also have larger surface to volume ratio, smaller size, and other chemical properties which maximizes the range of potential active sites for a variety of chemical reactions [24]. Given their remarkable abilities like as adsorption capacity, high surface area, durability and crystallinity, as well as their efficiency in storage and stability, nanomaterials may be employed in a wide variety of ways to fulfil the fast-expanding global energy needs. A broad range of applications for natural polymers in bioenergy production include cellulase synthesis and thermal stability, biomass pretreatment and waste management, as well as sugar and biohydrogen generation etc. The usage of NPs such as Fe3O4 and Fe3O4/alginate, for example, Srivastava et al. [25] founded in the study, when enzymatic hydrolysis was performed on a biomass sample, the hydrolysis proficiency and cellulase production improved by 35% and 40%, respectively. When heated to 80°C for 7 hours, a similar set of authors discovered that nickel cobaltite (NiCo2O4) NPs boosted cellulase production by 40% while also enhancing thermal stability [26]. Srivastava et al. [27] conducted research on zinc oxide-based NPs for the pH and thermal stability of crude cellulase and their results showed thermal stability was improved at pH value of 10.5 with an average temperature of 65°C for 10 hour. Similarly in another study, Dutta et al. [28] discovered that calcium hydroxyapatite-based NPs increased the thermal stability of the system and increased the synthesis of d-xylose by 35%. It has been shown that activated NPs may activate both xylanases and cellulases, which are then used only at increased temperatures of up to 80°C. Additionally, it was noted that using calcium in a NPs form increased the thermostability and xylanase and cellulase enzymatic activities.
The NPs have been used in a wide range of ways, and each has brought about significant changes in its respective field [29]. Magnetic nanoparticles (MNPs) have been the most widely used nanoparticles in bioenergy production in contrast to other NPs because their magnetic properties allow for easy recovery and binding to multiple target compounds, as well as their exceptional biodegradability, ease of synthesis and short cytotoxicity to cells. It has previously been reported that the lipase may be reused four times without any loss of activity or performance, and this was confirmed by Xie and Ma [30] who employed magnetic Fe3O4 NPs treated with (3-aminopropyl) triethoxysilan to support the lipase immobilization. Some additional MNPs have been employed for the immobilisation of enzymes, including TiO2 for the hydrolysis of lignocellulosic material to produce industrial products [31], a combination of TiO2 and ZnO for biodiesel production through palm oil transesterification process [32], and manganese dioxide for bioethanol production. Furthermore, it has been shown that the use of NPs or their micro emulsions has a hydrolytic impact that is equivalent to that obtained with chemical pretreatment in the processing of LCB.
Perfluoroalkylsufonic acid and alkylsulfonic acid functionalized magnetic NPs treatment of wheat straw resulted in a 46 percent increase in sugar production when compared to the control treatment (35 percent). Perfluoroalkylsufonic and alkylsulfonic acid might have stabilised and dispersed hemicellulose hydrolysis with an acidity level comparable to sulphuric acid solutions [33]. Using magnetic nanoparticles has a number of advantages, according to the authors, the most notable of which is their remarkable ability to pretreat lignocellulosic biomass with small amounts of material while remaining recyclable for future applications. The combined impact of alkaline pretreatment with magnetite NPs on rice straw for biogas generation was also identified [34], and this combination effect may be investigated further in a future research of pretreatment. Biogas production has increased dramatically after the deployment of MNPs, with outputs of biogas and methane increasing by 100 and 129 percent, respectively. NPs were also used to boost sugar production even during pretreatment stage. Hemicelluloses were transformed by 61 to 66 percent when sulphuric acid was mixed with perfluoroalkylsufonic and alkylsulfonic acid nanomaterials at 50 and 400-fold acid concentrations, respectively. Other investigations have found that NPs of trace elements with organic sources including bioslurry, have strong coercivity and paramagnetic characteristics make them ideal for biogas and methane production [35]. Adding magnetite (Fe3O4) NPs at concentrations of up to 20 mg/L, according to Abdelsalam et al.[36], decreased the time required to achieve the highest levels of biogas and methane production.
Biomass derived from lignocellulosic materials has shown promise as a solution to the problem of environmental safety. It is a major source of energy generation, with a massive output all over the world. The supply of lignocellulose biomasses in the United States alone is expected to reach 450 million dry tonnes per year by 2030, which is equivalent to an annual production capacity of 67 billion gallons of ethanol [37]. The multistep process of converting LB into fermentable sugars and bioenergy needs precise technology from the beginning to the end.
Converting LCB to significance products requires few steps including: a), LCB must be pretreated in order to break down the biomass configuration and sort it appropriate for the enzymatic process; b), then the components must be hydrolysed by enzymes in order to release sugars for fermentation; and c), finally the sugars must be fermented in order to convert monomer sugars into biofuel or other significant products, depending on the application. Lignocellulose biomasses may be used to produce bioenergy in a variety of ways, as seen in Figure 4. You may turn lignocellulosic biomass into biochemical products utilizing a number of pretreatment procedures, such as those using a combination of the three. Conventional procedures, on the other hand, are prohibitively expensive, time consuming, and limited in their use. As a result of the introduction of nanotechnology new and innovative technique that is both cost-effective and ecologically benign these limitations have been overcome. The use of nanotechnology as a pretreatment technique is a viable option [38].
Treatment methodology of lignocellulose in various steps
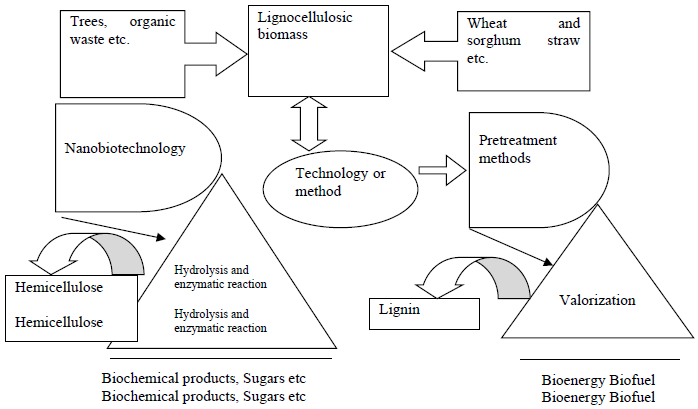
To begin the bioenergy conversion process, LCB is pretreated, which breaks down the complicated structure of LCB to release the polymers. Treatment of LCB is an essential stage, and the most effective pretreatment procedure must be carefully chosen. Pretreatment procedures that are successful, on the other hand, must fulfil a number of criteria, which include the following elements: It is necessary to achieve improvements in sugar production from LCB or the capacity to manufacture sugars through hydrolysis, as well as: 2) strong resistance to degradation or depletion, 3) restriction of the accumulation of by-products that impede further hydrolysis, cost-effectiveness of the technology [39] and fermentation processes. The chemistry of biomass may be improved at a molecular level by using nanomaterials for pretreatment [40]. Reusability may be improved by utilizing magnetic nanoparticles, which are easier to remove from reaction medium and hence more cost effective as a whole. As with chemical pretreatment, the hydrolytic action of nanoparticles or their microemulsions has been shown for LB processing [41]. The increasing needs for energy around the world are satisfied by the utilisation of fossil fuels and the application of appropriate technology, such as nanotechnology, which provides a viable solution to the problems associated with conventional pre-treatment procedures [42]. Wheat straw treated with acid-functionalized PFS and AS MNPs generated 46% more sugar than the control (35 percent) in research conducted by various researchers. Some studies claim that adding NPs to AS and PFS acids resulted in the stability and allocation of hemicellulose hydrolysis, which has an acid strength comparable to sulphuric acid solutions. The use of MNPs in biogas production has exhibited a significant progress, with yields of biogas and methane increasing by 100 and 129 percent, respectively, when compared to previous studies. It has been shown that NPs may boost sugar production during the pretreatment stage. Yellow poplar sawdust was treated at 175°C to yield 96 percent (w/w) hemicellulose using 0.8 percent (w/v) sulfuric acid to yield 96 percent (w/w) hemicellulose. When sulfuric acid was used in conjunction with PFS and AS nanomaterials, hemicelluloses were transformed by 61 to 66 percent, respectively, at 50- and 400-fold acid concentrations.
NSHA pretreatment (nano-shear hybrid alkali) is an alternative new technique to LCB pretreatment that is based on nanotechnology. As part of this approach, high-speed shear and chemical reagent synergy are combined with a milder heat action to get the desired results. In most cases, this reaction is carried out in a special reactor called as a nanomixing reactor. It is possible to convey the high shearing work axis to the nanostructure, which allows for efficient lignin removal while also exposing the cellular biomass components in a short period of time, resulting in higher productivity. A maize stover pretreatment method based on the NSHA approach was developed by Wang and Lee [43]. The higher shear rate of synergic effects shows that introducing high shearing into current pretreatment processes, as well as a wide range of other chemical products, might be beneficial [44]. Poly diallyldimethylammonium chloride) (PDAC) is used as an additive in another study of the NSHA pretreatment of corn stover. It changes the cellulosic surfaces and stabilizes lignin. Lignin and PDAC polyelectrolyte form a globular aggregate, which changes the shape of the cell wall. Polyelectrolyte is used in a way that reduces the amount of chemicals used [45]. As a result, there are few reports on biomass pretreatment using NPs at this time, owing to the fact that nanotechnology is still relatively new in biomass refineries.
Previous studies concluded that, using nanoparticles or nanomaterials to break down lignocellulosic biomass can be done in two ways: by putting different enzymes on the nanomaterials by using any source (food waste or agriculture waste) [46], or by using nanoparticles that have been modified. In the process of making biofuel, enzymatic hydrolysis is a very important step. However, the stability of the enzymes used in the process is very important. Nanotechnology is used in this step because it can help to keep these biocatalysts in place including enzymes. Compared to other potential substrates for immobilised enzymes, magnetic nanoparticles offer several benefits, including a larger surface area, which allows for a higher enzyme loading, decreased mass transfer resistance, reduced fouling effect, and selective, nonchemical separation from the reaction mixture via an applied magnetic field [47]. By combining cutting-edge nanotechnology and biotechnology, the promising new field of nanobiocatalysis has great promise for enhancing enzyme activity, stability, capability, and engineering performances in bioprocessing [48]. This shows that nanobiotechnology is always making new and interesting discovery. When enzymes are immobilized, they can be used again and again. This makes enzyme immobilisation a key tool for the development of second-generation biorefineries. Significantly higher enzyme activity has been achieved through the creation of new nanobiocatalysts in recent years [49]. There is a spacer between nanomaterials and enzymes that makes them more specific to substrates and more flexible. Furthermore, Enzymatic hydrolysis is seen as a potential way to producing bioethanol from a wide variety of lignocellulosic biomass to meet the world's growing energy needs [50]. There are four main types of immobilization strategies [51] including covalent bonding, entrapment/encapsulation, cross-linking and adsorption. These strategies are based on how the enzymes interact with each other. As an example, the adsorption method is thought to be a cheap and simple way of getting the job done. The main disadvantages of this method are the weakening of interactions and the risk of enzyme leakage when the pH, temperature, or polarity of the medium changes. Because covalent bonding makes enzymes more stable and the bonds made are strong enough to keep enzymes from leaving, it is often used for enzyme immobilisation. Lignocellulose hydrolysate contains inhibitors, making the encapsulation process more effective since it may create a microenvironment that prevents enzymes from being exposed to adverse conditions. Cross-linking is a different kind of immobilisation method because it creates enzyme aggregation, or "cross-linking enzyme aggregates." This process is called "cross-linking" (CLEAs). When it comes to enhancing the qualities of immobilised enzymes for use in industry, CLEAs offer practical and effective methods [52]. Nanofibers, NPs, and organic metal frames are all used to immobilize enzymes in a different way. All of them do the same thing. The MNPs, nickel NPs and iron NPs, as well as other metal oxide NPs, have all been employed as transporters for enzyme immobilisation.
Adsorption process was used in a study by Tang et al. [53] to put glucose oxidase on a carbon nanotube electrode that had platinum NPs on it. This made the enzyme stick to the electrode. Immobilization gets better when nanotubes are used. In general, enzymes were immobilized on nanoparticles or other support materials in order to improve their functionality (Table 1). Microbiological uses of immobilisation technology may be expanded thanks to the exceptional qualities of immobilised cells, such as enhanced metabolic activity, enhanced growth rate, plasmid stability, and protection from toxicity [54]. It has been shown that immobilisation may prevent the occurrence of numerous inhibitory effects. For the reason that its active site is blocked from being approached by an inhibitor, it has this effect. People may have become more tolerant to inhibitors as a result of this. There is still a lack of consolidated evidence about how immobilisation affects microbial physiology [55].
Applications of NPs in the presence of substrate enhance the energy production
Sr. No. | Substrate | Nanoparticle | Products | Reference |
---|---|---|---|---|
1 | LCB | Magnetic cross-linked cellulose | Enzymes retained 74% activity | [56] |
2 | LCB | Zinc oxide NPs | Enzymes retained 53% at pH 10 | [27] |
3 | LCB | Magnesium oxide | Enzymes stable 1.92-fold | [57] |
4 | LCB | MNPs | 19 g per litter glucose | [58] |
5 | Leaves | Chitosan-coated | Enzymes retained 80% | [59] |
6 | Cellulose | Zinc magnetic NPs | - | [60] |
7 | Cellulose | Magneto graphene | Enzymes retained 80% | [61] |
8 | LCB | Multiwall carbon | - | [62] |
LCB, lignocellulose biomass, NPs, Nanoparticles, MNPs, Magnetic nanoparticles
Cellulase enzymes that can withstand high temperatures are important for large-scale industrial lignocellulosic operations because high temperatures speed up the process of breaking down biomass. It's one of the main advantages of using immobilized cellulases for large-scale production because they are more stable at high temperatures. Another benefit of an open porosity shape is that it makes it easier for a substrate to get to the enzyme. The chitosan- and glutaraldehyde-functionalized, glutaraldehyde-activated, as-prepared chitosan/magnetic porous biochar supported the covalent immobilisation of cellulase [63]. This is because it gives the enzyme more surface area.
Research and development to increase biomass's value has been ongoing for decades, but commercial lignocellulose biomass production for the production of biofuels, such as biodiesel, remains a technical and economic challenge to overcome. Using nanotechnology, it is possible to create a highly productive microbiome capable of producing sustainable biofuels by adding a novel metabolic route into its structure. It has been stated that the use of nanomaterials or NPs during the fermentation process might lower the overall cost of converting LCB to biofuel. Enzyme immobilisation has been identified as a possible method for LB hydrolysis, despite the prevalence of acid/enzyme catalysts in this process [64]. Nanotechnology has been utilized to improve the efficiency and cost-effectiveness of bioethanol production, as well as to develop a number of new technologies. A number of enzymes, including cellulases, cellobiases, and -glycosidase, were immobilized on different NPs and used in the bioethanol fermentation process. It has been shown that this procedure has superior properties when compared to free enzymes. Increases in enzyme output and new methods for prolonging their stability are two pressing needs of the biotech sector [65]. In addition to improving the biostability and effectiveness of the immobilised enzyme, Alnadari et al. [66] found that the use of a thermostable chitin-binding domain during the immobilization of glucosidase from Thermotoga maritima on chitin MNPs produced a low-cost Galacto-oligosaccharide. When executing the continuous process, one clear advantage of immobilisation is the ability to quickly retrieve and recycle enzymes, as well as the ability to recover product free of enzymes and with minimum inhibition of the end product throughout the process (Table 2).
NPs applications improve biofuel production
S.No. | Materials/stocks/sources | Nanoparticle | Product | References |
---|---|---|---|---|
1 | Weeds | Nickel and cobalt | Biogas and biodiesel | [67] |
2 | Potato peels | NiO | Bioethanol | [68] |
3 | Sugarcane straw | Fe3O4 | Biohydrogen | [69] |
4 | Sorghum sweet stover | Fe3O4 and SiO2 | Biohydrogen | [70] |
5 | Grasses straw | FeO | Biohydrogen | [71] |
6 | Algal biomass | - | Biohydrogen | [72] |
7 | Fermentation | Nickel | Biohydrogen | [73] |
8 | Industrial effluent | NiO and CoO | Biohydrogen | [74] |
9 | Sugarcane juice | Magnetite | Biohydrogen | [75] |
10 | Sunflower oil | CaO | Biodiesel | [76] |
11 | Chenopodium album | Ni and Co | Biodiesel | [77] |
A critical role in the synthesis of biohydrogen is played by the hydrogenase enzyme during the dark fermentation process. Biohydrogen is quickly becoming one of the most popular renewable energy options due to its high conversion efficiency to usable power and low pollution output. Currently, various methods are in use to convert lignocellulosic biomass into biohydrogen [78]. The use of nanoparticles and materials in the biohydrogen production process is regarded to be an innovative approach of increasing metabolic activity in the process. When nanotechnology is used to promote ferredoxinoxidoreductase activities, it may result in greater electron transfer rates and increased catalytic efficiency, which are both beneficial.
Separately, the researchers reported that sugarcane feedstock was hydrolysed under ultraviolet light in a combination of diluted H2SO4 and TiO2 NPs. Sugar and hydrogen output improved by 260 and 127 percent, respectively, due to this method [79]. The majority of the study focused on the contact among nanomaterials and enzymes that happens during the process of increasing the electron transfer rate and, therefore, increasing biohydrogen production and enzyme productivities. It is expected that after interacting with nano biocatalysts, LCB would be significantly more responsive to enzyme treatment for substrate hydrolysis into sugars and consequent bacterial fermentation for renewable energy production and other bio-based goods.
Biorefineries utilize biomass to power complicated processing systems that turn the biomass into a diverse spectrum of fuels, bio-based chemicals-based products. Multiple lignocellulosic biomass techno-economic studies have concluded that complexity is the determining element in the expansion of the lignocellulosic biomass-based sector of the economy. If the kind or complexity of lignocellulosic biomass were to change, conversion at the pilot-scale would be halted, perhaps leading to the shutdown of the project or plant. Availability, transport, and conversion cost of biomass also play significant roles in commercialization [80]. Environmentally friendly energy and chemicals are produced in large quantities by second-generation biorefineries, which hold enormous potential for the future. They generate energy by the utilization of feedstock such as lignocellulosic biomass, non-edible crops, and waste materials such as sawdust, black liquor and straw, among other items. Integrating several biorefineries increases feedstock use by generating the largest quantity of value-added commodities [81] while producing the least amount of garbage by changing it into lesser value-added products. This boosts output while decreasing overall costs. Pretreatment of lignocellulosic materials, which can be quite refractory, is necessary for the sugar-based biorefinery platform in order to improve subsequent processing via enzymatic hydrolysis and for other downstream unit activities [82]. Because of the declining need for paper, for example, there is now a tendency in the wood sector to convert pulp mills into integrated biorefineries to meet the growing demand for renewable energy. A pulp mill's black liquor is utilized for wood or paper processing, and the lignin recovered from this liquor is used to make paper. As part of its bio-economy policy, the EU announced a bio-refinery strategy in 2012, with the goal of building sustainable biorefineries that are based on cost-effective lignocellulose biomass conversion into bioenergy and bioproducts. By 2030, the European Union hopes to contribute 25 percent of the energy used in transportation using biofuels supplied from biorefineries. Many European and Asians scientists are seeking to identify strategies to minimize the cost of processes, and they are not alone in their efforts in this direction.
Lignocellulosic biomass may be valued by turning it into usable products in one of three ways: First, biomass may be split into its constituent parts, with the constituent parts then being processed into goods and energy sources. First, lignin is removed from biomass by means of a process known as solvolysis, which involves the use of solvents. This is followed by depolymerization and stabilization using redox catalysts, which are then removed from the biomass. A by-product of this fractionation is the production of phenolic units and monolignol, which may then be utilized as feedstock for the production of value-added goods such as aromatic chemicals, polymers, bio-based fuels, and pharmaceuticals. Second, biomass may be partly degraded, after which it can be separated and upgraded to make useful. For example, pyrolysis may be used to generate bio-oil, which can then be enhanced further by enhancing the fuel qualities. Third, gasification entirely decomposes biomass into syngas, which is used to power vehicles. In the chemical industry, syngas is used as a precursor for hydrogen generation, and it may also be turned into fuels and organic compounds using the FischerTropsch synthesis process [83]. Biomass gasification, on the other hand, is not economically feasible due to the presence of methane and tar in syngas complexes. According to the scientist, catalytic biomass gasification is becoming increasingly popular across the world, increasing gasification efficiency by roughly 10%. Alternatively, the gas produced might be instantly combusted to generate energy. As a result of climate change, societal pressures, and new policy and law, many sectors are in the midst of a decarbonization process that involves a significant transition away from a fossil fuel economy and towards a biomass-based one [84].
The combined burning of biomass and coal is a well-known way to make both power and heat, and many plants are already in use around the world to show that it works. For the cost-effective and long-term conversion of LCB into value added products, integrating biorefineries is important. Biorefineries deal with issues like low efficiency and high operational costs, as well as high energy consumption in different processes that turn lignocellulosic biomass into value-added products. Many integrated biorefineries are being developed in various regions of the world. However, as previously stated, there are a few constraints that limit the alternatives and render this strategy ineffective on a broad scale. There are several factors that make scaling up biorefineries difficult, including high capital costs, a restricted biomass supply chain, and bottlenecks in each conversion phase.
Because of their resistance to chemical and biological degradation, the LCB converters are referred as "biomass resistant." A number of characteristics, including cellulose crystal structure, lignification degree, and structural heterogeneity and ambiguities of cellular components, contributes to biomass recalcitrance, which must be addressed in order to exploit the full potential of lignocellulosic feedstock. The most alluring renewable bioenergy sources are agricultural wastes like lignocellulosic materials (LM), which are abundant in nature. Biogas generation from LM by anaerobic digestion has been the subject of much research [85]. In this regard, pretreatment is a vital step in the biorefinery process and should not be overlooked. Furthermore, biomass from other sources, such as crop biomasses and organic wastes, should be subjected to similar treatments in order to alleviate the energy crisis. Various pretreatments that are being used as discussed in Figure 5.
Various pretreatment methods responsible for biomass conversion
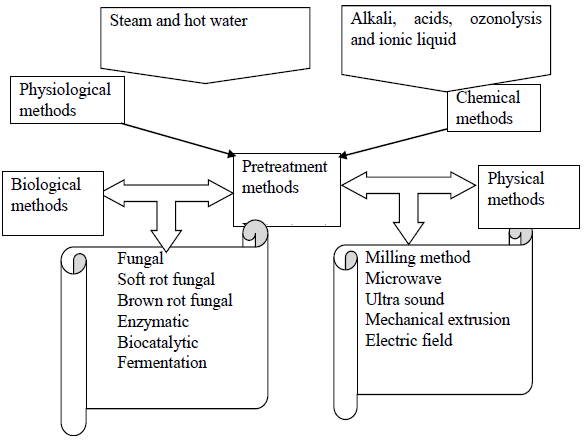
Pretreatment processes have been developed over the last few decades to improve the digestibility of LCB in order to improve the yield [86]. These treatments may be classified as shown in Figure 4 to aid in the classification of LCB. Because the quantity of cellulose, hemicellulose, and lignin vary depending on the kind of LCB used, the most convenient pretreatment process must be selected from among the multiple available options. Low-cost, abundant, renewable and a type of natural resource, LCB includes a variety of agro-industrial biomass such as sugarcane bagasse, rice and wheat straw, maize stover, and switchgrass [87]. The essential pretreatment processes and their influence on the separation of the complex components of varied lignocellulosic sources are discussed in detail in the following sections.
The physical preparation of LCB is required prior to the use of any other pretreatment techniques. It is generally used to reduce particle size, which leads in a rise in surface area, as well as a reduction in the degree of polymerization and crystallinity, among other benefits. Different biomass feedstocks have prompted the development and testing of unique pretreatment systems. The breakdown of sugars and the development of inhibitors during pretreatment is a major cause for worry when using pretreatments [88]. Therefore, the procedures that follow become more effective and less difficult to manage.
Conventional procedures for chemical and physical pretreatments need the use of costly chemicals, sophisticated equipment, and a lot of electricity. When it comes to the treatment of lignocellulosic material, biochemical pretreatment is preferred since it requires the employment of living bacteria, and this procedure is both more ecologically friendly and energy efficient than chemical pretreatment. Currently, discussion of the many pretreatment methods used in the generation of bio-hydrogen, bio-methane, bio-ethanol, bio-methanol, bio-butanol, and bio-diesel [89]. Cellulolytic and hemicellulolytic characteristics are possessed by a diverse range of microorganisms in nature, some of which are abundantly distributed. All three types of fungus are known for their capacity to extract lignin and hemicellulose from wood while having minimal effect on the cellulose content of the wood. This is due to the presence of lignin-degrading enzymes in white rot, including as peroxidases and lactases, which enable it to damage the fibre in the wood. Hatakka [90] conducted research on the pretreatment of wheat straw. The findings revealed that Pleurotus ostreatus converted 13 percent of wheat straw into sugars over the course of 5 weeks, but Phanerochaete sordida and Pycnoporus cinnabarinus converted virtually the same amount of wheat straw into sugars during a shorter period of time.
Biofuels can be made from a wide variety of lignocellulosic biomasses, including wheat straw, sugarcane straw, corn stover and specific waste materials. Thus, scientists are using a wide range of lignocellulosic waste material with more recent technologies including biotechnological ones with ecological and economic benefits like limiting fossil fuel usage, reducing harmful emissions and establishing a suitable platform for a diversity of microorganisms that could be helpful in renewable energy production. Sustainable bioenergy (biogas and biodiesel) has been generated due to biotechnology and represents an attractive option for the disposal of organic waste. Greenhouse gas emissions and waste management issues are reduced when biomass is produced using organic waste and carbon dioxide from gases. This further improves the sustainability of biofuels of the third generation [91]. The majority of the organic fraction consists of polymers that are readily biodegradable by microorganisms, and these are primarily carbohydrates. Furthermore, nanotechnology is a practical method for converting biological waste into useful products (biodiesel). Hydrogen gas is a promising energy option since it may be used indefinitely as a clean fuel for a wide variety of purposes. TiO2 and cadmium sulphide nanostructures are two examples of semiconductor nanomaterials that have been explored as potential catalysts for splitting water into oxygen and hydrogen. Some of the nanomaterials being researched for hydrogen storage and transportation have high hydrogen capacity and minimum deterioration during hydrogenation, and these include nano-structured carbons, metal-organic frameworks, and polymers. Energy Breakthroughs in nanotechnology could bring new energy-security and energy-supply solutions. Rice University Texas, identified numerous areas where nanotechnology can launch cheap, efficient, and environmentally beneficial technologies. Nanotechnology has benefits in solar energy, wind energy, fusion reactors, clean coal, fission reactors, hydrogen production, storage, shipping, and transportation, fuel cells, and batteries.
The term "green chemistry" refers to the practice of creating substances without negative effects on human or environmental health [92], and this field has been acknowledged as a distinct branch of chemistry for over 20 years. When combined with the fundamentals of green chemistry, this novel idea has the potential to greatly improve the development of environmentally friendly nanomaterials and establish new benchmarks in this area. Green techniques of manufacturing of nanoparticles from plants and microorganisms have been reported by scientists [93]. However, the hazardous consequences of NPs on the environment and health are a hurdle that green nanotechnology must overcome. Despite the promising potential of green synthesis nanomaterials in fields including energy storage, nano sensing, and nanomedicine, environmental remediation [94], there is more work to be done in assessing the risks and benefits of this emerging field of nanotechnology. By 2030, there will be 450 million dry tonnes of these biomasses available in the United States alone, enough to generate 67 billion gallons of ethanol per year. According to a European Union report issued in 2021, biomass from diverse sources (plants, trees, and organic wastes) provided 60% of total bioenergy. In order to address environmental changes, rising energy needs, and health difficulties on a global scale, the concept of a "green economy" has entered the discussion [95]. Nanotechnology provides novel, environmentally friendly approaches to producing cutting-edge devices and materials with tailored physical and chemical properties; the concept of green-economy may bear a significant responsibility in achieving a healthy equilibrium between the economic and environmental spheres by generating more green-jobs, improving resource management, and fostering increased expansion of vital economic sectors.
These days, recycling and energy efficiency are more important than ever before because of the state of the environment and the economy. In order to convert garbage into useful energy, scientists and engineers employed and created a wide range of novel technologies. The process of converting garbage into fuel for use in producing energy takes several forms. In recent years, one of the most promising strategies for advancing renewable energy has been the environmentally responsible harvesting and conversion of biomass waste into chemical fuels. The process of converting biomass into usable energy can be done using a number of different technologies. These two broad methods, thermochemical and biochemical conversion, are used in the process of converting waste biomass to energy alongside the transesterification approach. By contrast to biochemical conversion, which makes use of microbes or enzymes to transform biomass or waste into usable energy, thermochemical conversion involves the degradation of organic components in the biomass by means of heat. Recent studies into bioenergy conversion using various approaches are summarized in Figure 6.
Various emerging technologies or methods used in energy production from different source. This figure has been regenerated for the present manuscript. Yield related data were obtained from the source:[96], [97].
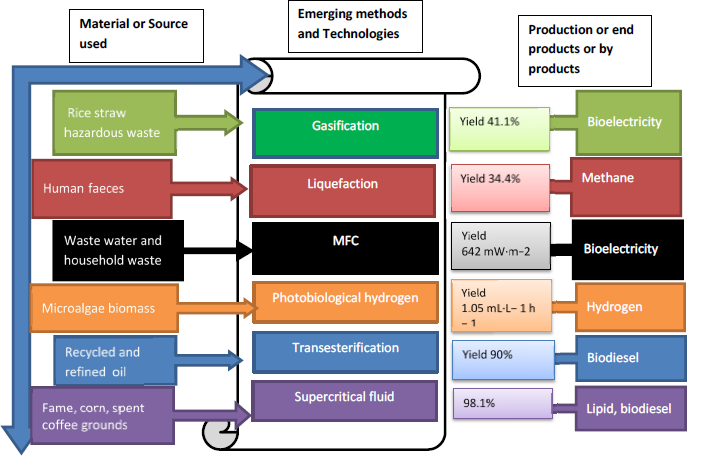
Renewable feedstock may be used to create bioelectricity [98], another type of bioenergy, in addition to biofuel generation. Biomass energy is a possible alternative energy source that may be derived from agricultural and forest resources. The major way of creating bioelectricity is by the combustion of lignocellulose fuel derived from agricultural commodities and wastes, plantation forests, sawmill leftovers, and natural forests. Researchers investigated the potential of creating bioelectricity from biomass in order to minimize greenhouse gas emissions. This study found that lignocellulose from forestry and agricultural biomass may be utilised to generate power by direct combustion. The current forestry and agricultural production systems provide 15% of the country's power, but that figure might increase by 9% if first-generation technology is updated. In addition to having a major impact on national emissions, energy-generation systems were able to lower emissions by around 28% for electricity and 9% for national emissions.
Supercritical carbon dioxide (SC-CO2) is the solvent used in the supercritical fluid extraction process (SFE). The SC-CO2 extraction technique enhances the yield and selectivity profile of plant organic compound extraction. Furthermore, much study has been conducted on lipid extraction utilizing SFE from third-generation feedstock. Furthermore, lipids from milk were extracted using the supercritical anti-solvent fractionation approach (SAFT). Because of its two major components, water and an organic solvent that is miscible with water, the SAFT may be used in a wide range of scenarios. A supercritical solvent is used to extract the process's final solution.
Catalysts of three sorts are frequently used in the production of biodiesel: acid, base, and enzymes. Base-catalyzed transesterification, as opposed to acid-catalyzed transesterification, is frequently employed in industrial production because it delivers a higher FAME concentration fast under mild reaction conditions. Enzyme catalysts, on the other hand, are better for the environment and can produce high-quality items, but due to their slow reaction rate and high cost, they require more research and development to become economically feasible. The esterification and transesterification processes are widely utilised in the production of biodiesel. To utilize biodiesel in ordinary internal combustion engines, the lipid granules must be transformed to a less viscous state. As a result, much more studies needed under different temperature, humidity and concentrations that use enzymes that are at least partially active under normal plant growth and cell wall formation circumstances.
Improved yields of important metabolites or the ability to switch metabolite production have both been investigated as potential outcomes of genetically engineering microalgal strains. One goal of genetic engineering is to modify photosynthesis so that it can convert carbon dioxide directly into biofuels without first having to generate energy by constructing and decomposing proteins used for energy storage or cellular structure. In the Calvin cycle of photosynthesis, ribulose-1,5-bisphosphate combines with carbon dioxide to produce two 3-phosphoglyceric acid, building blocks for the production of glucose and other metabolites. Studies have been done to insert genes responsible for ethanol production, which would cause the 3-phosphoglyceric acid molecules to switch to ethanol synthesis (pyruvate decarboxylase and alcohol dehydrogenase). Methanogenesis was developed into a recombinant strain of the proteobacterium Rhodobacter sp. in one investigation. An anaerobe, the recombinant strain produced ethanol in the presence of light but not oxygen. Few studies in this field will be conducted on designing plants to accumulate cell wall-degrading enzymes that are sequestered to organelles or are only active at extremely high temperatures.
The use of catalysts is an additional viable option for pyrolysis oil upgrading. In order to improve the quality of pyrolysis oil, two techniques can be used. There are two methods for upgrading fossil fuels: in-situ improvement by integrated catalytic pyrolysis, and downstream improvement using metallic or bi-functional (hydrogenating and acidic) catalysts. The vapour generated during pyrolysis will undergo additional cracking within the catalyst pore to produce a molecule with a low molecular weight during the catalytic process.
Population and economic growth, especially in developing Asian countries, are expected to increase energy demand globally by 47% over the next 30 years, according to the Energy Information Administration [99]. By 2050, liquid fuel will account for 28% of global energy demand, while renewables will account for 27% [99]. Compared to 2020 levels, this presumes a 165% increase in renewables and a 36% increase in liquid fuel demand [100]. Due to its size, complexity, path dependency, and reliance on long-lived assets, the energy sector presents a unique challenge. Since the early 1960s, international gas markets in Europe have flourished thanks to oil-indexed long-term agreements that continue to regulate gas imports to the European Union today. However, the strategic costs for the corporations resulted from their failure to adapt to the changing business climate. This is a politically charged issue, but studies to now have ignored procedures within individual businesses in favour of examining them at the national and regional levels [101]. There has been a dramatic rise in energy demand worldwide as a result of rising populations and economies. This increase will be driven primarily by large emerging economies, which will be responsible for 90% of the growth in energy demand between now and 2035. There may be a threat to energy security if the number of people using energy continues to rise. More carbon dioxide and other greenhouse gases are emitted as more fossil fuels are used, which furthers global warming. Eighty-nine percent of the carbon dioxide emissions in the world in 2018 originated from burning fossil fuels and industries [102].
New ways of thinking and new systems will have to be implemented if we are having to achieve a sustainable energy future. This entails altering how energy is created, distributed, and used. Energy development must be sustainable if we are to meet our goals of improving living standards, expanding access to modern energy services, reducing energy waste, protecting the global environment, and ensuring stable energy supplies [103]. The environment and economics make recycling and energy efficiency more vital than ever. Scientists and engineers invented unique ways to transform trash into electricity [104]. Waste-to-energy conversion takes numerous forms. Forest, agricultural, municipal solid waste, and paper waste are only few of the industries whose byproducts make up the lignocellulosic biomass. An abundant organic polymer found in plants; LB is largely underutilization. Now a days developing countries producing feedstock for biofuel production from waste [19]. South and Southeast Asian countries face unique difficulties in protecting the environment, which hinders their progress towards sustainable development. Air pollution has become a major problem during the past two decades, especially in the most industrialized and highly populated countries [101]. A staggering 1x1010 metric tonnes of lignocellulosic biomass is produced every year, making it a tremendously large resource for the generation of energy. The demand for biomass-based goods is expected to rise worldwide during the coming decades. Biofuels and bio-based materials will increasingly drive future economies while also ensuring food security for a larger and more prosperous global population. By 2050, we need to have completely transitioned away from fossil carbon and towards a bioeconomy model, if we're going to meet the goals of the Paris Climate Change Agreement [105]. Cellulose and hemicelluloses are two components of the lignocellulose complex present in plant cell walls; after cellulose, lignin is the most common polymer [106]. There are considerable differences in the proportions of hemicellulose, cellulose, and lignin among the plant cell wall components known as the main wall, secondary wall, and middle lamella [107]. It is generally known that lignin significantly inhibits the hydrolysis of the polysaccharide components of plant cell walls, cellulose and hemicellulose, by microbial enzymes. Furthermore, lignin is produced commercially on a global scale as a byproduct of the paper making industry [108].
In the past, many pre-treatment strategies have been implemented, including physical, chemical, physicochemical, and biological techniques [109]. However, these techniques are time-consuming and costly, and can result in hazardous inhibitors. Nanotechnology, a recently developed alternative that is cost-effective, efficient, and environmentally friendly, offers a promising answer to the issues that have plagued traditional pre-treatment strategies. Nanotechnology is being widely used in many different fields, including the biofuel industry. Nanomaterials are used in the processes that rely on nanotechnology. Nanomaterials with magnetic characteristics are preferred [110], because they can be easily separated from the reaction liquid and reused, reducing costs. Many scientists have immobilized cellulase, hemicellulose, and ligninolytic enzymes on nanomaterials for application in enzymatic hydrolysis. Since these nanobiocatalyst may be recovered and reused again in hydrolytic reactions, their use as an enzyme immobilized functionalized magnetic nanomaterial would provide a game-changing alternative to current approaches. In order to save costs, it would be beneficial to recycle the nanobiocatalyst that contain the immobilized enzymes. Since acid isn't needed for every cycle, using acid-functionalized magnetic nano-catalysts reduces the intensity of pre-treatment procedures. Increased hydrolysis and sugar recovery would result from immobilizing sufficient amounts of acids and enzymes on the nanomaterials, which would be possible due to the high surface-to-volume ratio.
In spite of the much compensation of nanotechnology and nanomaterials in bioenergy production from LCB, there are a number of challenges that must be addressed before this technology can be extensively used. Among them are the following: For example, immobilization by covalent binding is a critical strategy for preventing enzyme leakage during a process, which is particularly important when solid materials are used as carriers for enzymes. However, too far, information on the contact between lipase and carrier has been sparse, and further research is required in order to fully use the potential of NPs in bioenergy production. It is not yet known how NPs induced toxicity in different things such as metal nanocatalysts, produce toxicity in living creatures, although their tiny size may interfere with human cell function. Asthma and other respiratory diseases are substantially worse when NPs, which are included in biofuel, are released into the atmosphere during combustion of fuel. Consequently, security valuation is often extreme significance and must be carried out with meticulous care in order to alleviate concerns regarding energy, safety, and environmental issues associated with the use of nanomaterials for biofuel production, among other applications. Currently, there is considerable concern about the current pretreatment strategy, which is viewed as being uneconomical biofuel production, like biomass hydrolysis, which downs an ample quantity of energy and is not cost effective. The use of microwave reactors for chemical processes may be investigated as a means of overcoming this restriction in order to shorten the reaction time and, as a consequence, to lower the amount of energy used during the reaction. The advancement of nanotechnology is also essential for the development of more versatile nano-based catalytic systems, as it will allow them to be used to process a broader range of biomasses while also retaining impurities that could be harmful to the bioenergy productional processes, such as alkali and acidic metals. There are significant challenges associated with LCB, their processing, and the use and exploitation of nanomaterials, but it is anticipated that bioenergy production will be accelerated, which could mark a watershed moment in the green fuel industry if these issues can be resolved.
According to the current review, nanobiotechnology has been shown to be an essential component of lignocellulosic biomass pretreatment. Nanomaterials may readily enter the cell wall of lignocellulosic biomass due to their tiny size. This enables them to interact with lignocellulosic material including cellulose, hemicellulose, and lignin, making these components more available for the enzymatic hydrolysis process that transforms carbs. There is an urgent need for clean and renewable energy sources as the globe heads into energy crises, and this is possible with modern information. A deeper comprehension of nanobiotechnology, together with careful preparation and planning for its application, has the potential to reduce and even eliminate the unintended consequences outlined above. Furthermore, due to their magnetic properties, magnetic nanoparticles can be used to effectively recover and reuse immobilised enzymes. Despite reports of nanomaterials' usefulness, no clear evidence of the nanoparticle's mechanism of action in the pretreatment of lignocellulosic biomass has yet been presented. As a result, further in-depth research is needed to investigate the efficacy of the nanomaterials. This literature evaluation finds that nanomaterials will play an essential role in the pretreatment of lignocellulosic biomass for long-term bioenergy generation. Pretreatment of lignocellulosic biomass would benefit greatly from the application of nanomaterials in the not-too-distant future.
Circular Economy Aspects of Lignin: Towards a Lignocellulose Biorefinery ,Renew. Sustain. Energy Rev. , Vol. 130 ,pp 109977 , 2020, https://doi.org/https://doi.org/10.1016/j.rser.2020.109977
, Hydrolysis of lignocellulosic materials for ethanol production: A review ,Bioresour. Technol. , Vol. 83 (1),pp 111 , 2002, https://doi.org/https://doi.org/10.1016/S0960-8524(01)00212-7
, - Oil consumption worldwide from 1998 to 2021, 2023, https://www.statista.com/statistics/265239/global-oil-consumption-in-barrels-per-day/
Recent Developments in Lignocellulosic Biofuels, a Renewable Source of Bioenergy ,Fermentation , Vol. 8 (4),pp 161 , 2022, https://doi.org/https://doi.org/10.3390/fermentation8040161
, A review on the pretreatment of lignocellulose for high-value chemicals ,Fuel Process. Technol. , Vol. 160 ,pp 196206 , 2017, https://doi.org/https://doi.org/10.1016/j.fuproc.2016.12.007
, - , , Biofuel and Biorefinery Technologies, 2018
The role of biomass and bioenergy in a future bioeconomy?: Policies and facts ,Environ. Dev. , Vol. 15 ,pp 334 , 2015, https://doi.org/https://doi.org/10.1016/j.envdev.2015.03.006
, Biofuels 2020: Biorefineries based on lignocellulosic materials ,Appl. Microbiol. Int. , Vol. 9 (5),pp 585594 , 2016, https://doi.org/https://doi.org/10.1111/1751-7915.12387
, The Expansion of Lignocellulose Biomass Conversion Into Bioenergy via Nanobiotechnology ,Front. Nanotechnol. , Vol. 3 ,pp 110 , 2021, https://doi.org/https://doi.org/10.3389/fnano.2021.793528
, A Fourier transform infra-red spectroscopic analysis of the character of hydrogen bonds in amorphous cellulose ,Polymer (Guildf). , Vol. 37 (3),pp 393399 , 1996, https://doi.org/https://doi.org/10.1016/0032-3861(96)82908-9
, Recent developments in pretreatment technologies on lignocellulosic biomass: Effect of key parameters, technological improvements, and challenges ,Bioresour. Technol. , Vol. 300 ,pp 122724 , 2020, https://doi.org/https://doi.org/10.1016/j.biortech.2019.122724
, Lignin from Micro- to Nanosize?: Applications ,Int. J. Mol. Sci. , Vol. 18 (11),pp 2367 , 2017, https://doi.org/https://doi.org/10.3390/ijms18112367
, Efficiency of Lignin Biosynthesis: a Quantitative Analysis ,Ann. Bot. , Vol. 91 (6),pp 673695 , 2003, https://doi.org/https://doi.org/10.1093/aob/mcg073
, Bioconversion of lignocellulose?: inhibitors and detoxification ,Biotechnol. Biofuels Bioprod. , Vol. 6 ,pp 110 , 2013, https://doi.org/https://doi.org/10.1186/1754-6834-6-16
, Lignocellulosic agriculture wastes as biomass feedstocks for second-generation bioethanol production?: concepts and recent developments ,3 Biotech , Vol. 5 ,pp 337353 , 2015, https://doi.org/https://doi.org/10.1007/s13205-014-0246-5
, - , , Sugarcane Straw and Its Cellulose Fraction as Raw Materials for Obtainment of Textile fibers and Other Bioproducts, 2015
Lignin recovery from rice straw biorefinery solid waste by soda process with ethylene glycol as co-solvent ,J. Taiwan Inst. Chem. Eng. , Vol. 126 ,pp 5056 , 2021, https://doi.org/https://doi.org/10.1016/j.jtice.2021.07.030
, Bioethanol, biohydrogen and biogas production from wheat straw in a biorefinery concept ,Bioresour. Technol. , Vol. 100 (9),pp 25622568 , 2008, https://doi.org/https://doi.org/10.1016/j.biortech.2008.11.011
, Underutilized Lignocellulosic Waste as Sources of Feedstock for Biofuel Production in Developing Countries ,Front. Energy Res. , Vol. 10 ,pp 121 , 2022, https://doi.org/https://doi.org/10.3389/fenrg.2022.741570
, Review on nanoparticles and nanostructured materials?: history, sources, toxicity and regulations ,Beilstein J. Nanotechnol. , Vol. 9 ,pp 10501074 , 2018, https://doi.org/https://doi.org/10.3762/bjnano.9.98
, Carbon-based nanomaterials as an emerging platform for theranostics ,Mater. Horiz. , Vol. 6 (3),pp 434469 , 2019, https://doi.org/https://doi.org/10.1039/C8MH00966J
, Dependence of Nanoparticle Toxicity on Their Physical and Chemical Properties ,Nanoscale Res. Lett. , Vol. 13 ,pp 121 , 2018, https://doi.org/https://doi.org/10.1186/s11671-018-2457-x
, Nanoparticles: Properties, applications and toxicities ,Arab. J. Chem. , Vol. 12 (7),pp 908931 , 2019, https://doi.org/https://doi.org/10.1016/j.arabjc.2017.05.011
, Nanomaterial Properties: Size and Shape Dependencies ,J. Nanomater. , 2012, https://doi.org/https://doi.org/10.1155/2012/180976
, Improved production of reducing sugars from rice straw using crude cellulase activated with Fe3O4/Alginate nanocomposite ,Bioresour. Technol. , Vol. 183 ,pp 262266 , 2015, https://doi.org/https://doi.org/10.1016/j.biortech.2015.02.059
, Effect of NickelCobaltite Nanoparticles on Production and Thermostability of Cellulases from Newly Isolated Thermotolerant Aspergillus fumigatus NS (Class: Eurotiomycetes) ,Applied Biochem. Biotechnol. , Vol. 174 ,pp 10921103 , 2014, https://doi.org/https://doi.org/10.1007/s12010-014-0940-0
, Application of ZnO nanoparticles for improving the thermal and pH stability of crude cellulase obtained from Aspergillus fumigatus AA001 ,Front. Microbiol. , Vol. 7 ,pp 514 , 2016, https://doi.org/https://doi.org/10.3389/fmicb.2016.00514
, Improved production of reducing sugars from rice husk and rice straw using bacterial cellulase and xylanase activated with hydroxyapatite nanoparticles ,Bioresour. Technol. , Vol. 153 ,pp 269277 , 2014, https://doi.org/https://doi.org/10.1016/j.biortech.2013.12.016
, Review on Recent Progress in Magnetic Nanoparticles: Synthesis, Characterization, and Diverse Applications ,Front. Chem. , Vol. 9 ,pp 629054 , 2021, https://doi.org/https://doi.org/10.3389/fchem.2021.629054
, Immobilized Lipase on Fe3O4 Nanoparticles as Biocatalyst for Biodiesel Production ,Energy Fuels , Vol. 23 (3),pp 13471357 , 2009, https://doi.org/https://doi.org/10.1021/ef800648y
, - Immobilization of cellulase on TiO2 nanoparticles by physical and covalent methods: a comparative study, 2014, http://nopr.niscpr.res.in/bitstream/123456789/29326/1/IJBB, 51(4) 314-320.pdf
Biodiesel synthesis by TiO2ZnO mixed oxide nanocatalyst catalyzed palm oil transesterification process ,Bioresour. Technol. , Vol. 150 ,pp 5559 , 2013, https://doi.org/https://doi.org/10.1016/j.biortech.2013.09.087
, Acid-Functionalized Nanoparticles for Pretreatment of Wheat Straw. ,J. Biomater. Nanobiotechnol. , Vol. 3 (2),pp 342352 , 2012, https://doi.org/https://doi.org/10.4236/jbnb.2012.33032
, Synergistic effect of alkaline pretreatment and magnetite nanoparticle application on biogas production from rice straw ,Bioresour. Technol. , Vol. 275 ,pp 288296 , 2019, https://doi.org/https://doi.org/10.1016/j.biortech.2018.12.051
, Comparison of nanoparticles effects on biogas and methane production from anaerobic digestion of cattle dung slurry ,Renew. Energy , Vol. 87 (1),pp 592598 , 2016, https://doi.org/https://doi.org/10.1016/j.renene.2015.10.053
, Influence of zero valent iron nanoparticles and magnetic iron oxide nanoparticles on biogas and methane production from anaerobic digestion of manure ,Energy , Vol. 120 ,pp 842853 , 2017, https://doi.org/https://doi.org/10.1016/j.energy.2016.11.137
, - https://www1.eere.energy.gov/bioenergy/pdfs/billion_ton_update.pdf USA, 2011,
Enzyme Immobilization as a Strategy towards Efficient and Sustainable Lignocellulosic Biomass Conversion into Chemicals and Biofuels: Current Status and Perspectives. ,Sustain. Energy Fuels , Vol. 5 (17),pp 42334247 , 2021, https://doi.org/https://doi.org/10.1039/D1SE00747E
, New Trends in Application of Nanotechnology for the Pretreatment of Lignocellulosic Biomass. ,Biofuels, Bioprod. Biorefining , Vol. 13 (3),pp 776788 , 2018, https://doi.org/https://doi.org/10.1002/bbb.1965
, Emerging role of nanobiocatalysts in hydrolysis of lignocellulosic biomass leading to sustainable bioethanol production ,Catal. Rev. Sci. Eng. , Vol. 61 (1),pp 126 , 2019, https://doi.org/https://doi.org/10.1080/01614940.2018.1479503
, - , , in Nanotechnology for Bioenergy and Biofuel Production, 2017
Nanobiotechnological advancements in lignocellulosic biomass pretreatment ,Mater. Sci. Energy Technol. , Vol. 3 ,pp 308318 , 2020, https://doi.org/https://doi.org/10.1016/j.mset.2019.12.003
, Fast and Efficient Nanoshear Hybrid Alkaline Pretreatment of Corn stover for Biofuel and Materials Production ,Biomass and Bioenergy , Vol. 51 ,pp 3542 , 2013, https://doi.org/https://doi.org/10.1016/j.biombioe.2012.12.037
, - , , in Green Chemistry and Sustainable Technology, 2017
- Acid-functionalized nanoparticles for biomass hydrolysis, 2013, https://www.proquest.com/openview/1546d1eda0664f821f0bf7a9b622b949/1?pq-origsite=gscholar&cbl=18750
Nanomaterial conjugated lignocellulosic waste: cost-effective production of sustainable bioenergy using enzymes ,3 Biotech , Vol. 11 (11),pp 480 , 2021, https://doi.org/https://doi.org/10.1007/s13205-021-03002-4
, - , , Enzyme Stabilization and Immobilization, 2011
Nanobiocatalyst Advancements and Bioprocessing Applications ,J. R. Soc. Interface , Vol. 12 (102),pp 25392397 , 2015, https://doi.org/https://doi.org/10.1098/rsif.2014.0891
, Recent Advances in Enzyme-Nanostructure Biocatalysts with Enhanced Activity ,Catalysts , Vol. 10 (3),pp 338 , 2020, https://doi.org/https://doi.org/10.3390/catal10030338
, Comparative evaluation of free and immobilized cellulase for enzymatic hydrolysis of lignocellulosic biomass for sustainable bioethanol production ,Cellulose , Vol. 24 ,pp 55295540 , 2017, https://doi.org/https://doi.org/10.1007/s10570-017-1517-1
, An Overview of Techniques in Enzyme Immobilization ,Appl. Sci. Converg. Technol. , Vol. 26 (6),pp 157163 , 2017, https://doi.org/https://doi.org/10.5757/ASCT.2017.26.6.157
, Techniques for Preparation of Cross-Linked Enzyme Aggregates and Their Applications in Bioconversions ,Catalysts , Vol. 8 (5),pp 174 , 2018, https://doi.org/https://doi.org/10.3390/catal8050174
, Amperometric Glucose Biosensor Based on Adsorption of Glucose Oxidase at Platinum Nanoparticle-Modified Carbon Nanotube Electrode. ,Anal. Biochem. , Vol. 331 (1),pp 8997 , 2004, https://doi.org/https://doi.org/10.1016/j.ab.2004.05.005
, Metabolic Responses of Bacterial Cells to Immobilization ,Molecules , Vol. 21 (7),pp 958 , 2016, https://doi.org/https://doi.org/10.3390/molecules21070958
, Immobilized-cell physiology: Current data and the potentialities of proteomics ,Enzyme Microb. Technol. , Vol. 31 (3),pp 201212 , 2002, https://doi.org/https://doi.org/10.1016/S0141-0229(02)00073-X
, Novel Magnetic Cross-Linked Cellulase Aggregates with a Potential Application in Lignocellulosic Biomass Bioconversion ,Molecules , Vol. 22 (2),pp 269 , 2017, https://doi.org/https://doi.org/10.3390/molecules22020269
, Nanoparticle-induced enzyme pretreatment method for increased glucose production from lignocellulosic biomass under cold conditions ,J. Sci. food Agric. , Vol. 99 (2),pp 767780 , 2019, https://doi.org/https://doi.org/10.1002/jsfa.9245
, Immobilized Nanoparticles-Mediated Enzymatic Hydrolysis of Cellulose for Clean Sugar Production: A Novel Approach ,Curr. Nanosci. , Vol. 15 (3),pp 296303 , 2019, https://doi.org/https://doi.org/10.2174/1573413714666180611081759
, Cellulases immobilization on chitosan-coated magnetic nanoparticles: application for Agave Atrovirens lignocellulosic biomass hydrolysis ,Bioprocess Biosyst. Eng. , Vol. 40 ,pp 922 , 2017, https://doi.org/https://doi.org/10.1007/s00449-016-1670-1
, Suitability of magnetic nanoparticle immobilised cellulases in enhancing enzymatic saccharification of pretreated hemp biomass ,Biotechnol. Biofuels , Vol. 7 ,pp 112 , 2014, https://doi.org/https://doi.org/10.1186/1754-6834-7-90
, Immobilization of cellulase on magnetoresponsive graphene nano-supports ,J. Mol. Catal. B Enzym. , Vol. 90 ,pp 7686 , 2013, https://doi.org/https://doi.org/10.1016/j.molcatb.2013.01.025
, Immobilization of Aspergillus niger cellulase on multiwall carbon nanotubes for cellulose hydrolysis ,Bioresour. Technol. , Vol. 252 ,pp 7275 , 2018, https://doi.org/https://doi.org/10.1016/j.biortech.2017.12.082
, Preparation of chitosan/magnetic porous biochar as support for cellulase immobilization by using glutaraldehyde ,Polymers (Basel). , Vol. 12 (11),pp 2672 , 2020, https://doi.org/https://doi.org/10.3390/polym12112672
, Current Developments in Lignocellulosic Biomass Conversion into Biofuels Using Nanobiotechology Approach ,Energies , Vol. 13 (20),pp 5300 , 2020, https://doi.org/https://doi.org/10.3390/en13205300
, Enzyme immobilization?: an overview on techniques and support materials ,3 Biotech , Vol. 3 ,pp 19 , 2013, https://doi.org/https://doi.org/10.1007/s13205-012-0071-7
, Immobilization of β-Glucosidase from Thermatoga maritima on Chitin-functionalized Magnetic Nanoparticle via a Novel Thermostable Chitin-binding Domain ,Sci. Rep. , Vol. 10 (1),pp 112 , 2020, https://doi.org/https://doi.org/10.1038/s41598-019-57165-5
, Exploring the prospective of weeds (Cannabis sativa L., Parthenium hysterophorus L.) for biofuel production through nanocatalytic (Co, Ni) gasification ,Biotechnol. Biofuels , Vol. 13 ,pp 148 , 2020, https://doi.org/https://doi.org/10.1186/s13068-020-01785-x
, Impact of nanoparticle inclusion on bioethanol production process kinetic and inhibitor profile ,Biotechnol. Reports , Vol. 29 ,pp e00585 , 2021, https://doi.org/https://doi.org/10.1016/j.btre.2021.e00585
, Biohydrogen production via integrated sequential fermentation using magnetite nanoparticles treated crude enzyme to hydrolyze sugarcane bagasse ,Int. J. Hydrogen Energy , Vol. 47 (72),pp 3086130871 , 2022, https://doi.org/https://doi.org/10.1016/j.ijhydene.2021.08.198
, Optimal immobilization of Trichoderma asperellum laccase on polymer coated Fe3O4@SiO2 nanoparticles for enhanced biohydrogen production from delignified lignocellulosic biomass ,Fuel , Vol. 273 ,pp 117777 , 2020, https://doi.org/https://doi.org/10.1016/j.fuel.2020.117777
, Improving mechanisms of biohydrogen production from grass using zero-valent iron nanoparticles ,Bioresour. Technol. , Vol. 266 ,pp 413420 , 2018, https://doi.org/https://doi.org/10.1016/j.biortech.2018.07.004
, Tuning cationic/anionic dyes sorption from aqueous solution onto green algal biomass for biohydrogen production ,Environ. Res. , Vol. 216 ,pp 114522 , 2023, https://doi.org/https://doi.org/10.1016/j.envres.2022.114522
, Comparison of mesophilic and thermophilic dark fermentation with nickel ferrite nanoparticles supplementation for biohydrogen production ,Bioresour. Technol. , Vol. 329 ,pp 124853 , 2021, https://doi.org/https://doi.org/10.1016/j.biortech.2021.124853
, Impacts of nano-metal oxides on hydrogen production in anaerobic digestion of palm oil mill effluent A novel approach ,Int. J. Hydrogen Energy , Vol. 43 (5),pp 26662676 , 2018, https://doi.org/https://doi.org/10.1016/j.ijhydene.2017.12.108
, Biohydrogen production from sugarcane bagasse hydrolysate: effects of pH, S/X, Fe2+, and magnetite nanoparticles ,Environ. Sci. Pollut. Res. , Vol. 24 ,pp 87908804 , 2017, https://doi.org/https://doi.org/10.1007/s11356-017-8560-1
, Kinetics of sunflower oil methanolysis catalyzed by calcium oxide ,fuel , Vol. 88 (9),pp 15541562 , 2009, https://doi.org/https://doi.org/10.1016/j.fuel.2009.02.013
, Biofuels production from weed biomass using nanocatalyst technology ,Biomass and Bioenergy , Vol. 139 ,pp 105595 , 2020, https://doi.org/https://doi.org/10.1016/j.biombioe.2020.105595
, Biohydrogen Production from Lignocellulosic Biomass?: Technology and Sustainability ,Energies , Vol. 8 (11),pp 1306213080 , 2015, https://doi.org/https://doi.org/10.3390/en81112357
, Enhanced biohydrogen and subsequent biomethane production from sugarcane bagasse using nano-titanium dioxide pretreatment ,Bioresour. Technol. , Vol. 214 ,pp 670678 , 2016, https://doi.org/https://doi.org/10.1016/j.biortech.2016.05.007
, Lignocellulosic Biomass-Based Biorefinery: an Insight into Commercialization and Economic Standout ,Curr. Sustain. Energy Reports , Vol. 7 ,pp 122136 , 2020, https://doi.org/https://doi.org/10.1007/s40518-020-00157-1
, Integrated biorefinery processes for conversion of lignocellulosic biomass to value added materials: Paving a path towards circular economy ,Bioresour. Technol. , Vol. 343 ,pp 126151 , 2022, https://doi.org/https://doi.org/10.1016/j.biortech.2021.126151
, Pretreatment for biorefineries: A review of common methods for efficient utilisation of lignocellulosic materials ,Biotechnol. Biofuels Bioprod. , Vol. 12 ,pp 294 , 2019, https://doi.org/https://doi.org/10.1186/s13068-019-1634-1
, Application of FischerTropsch Synthesis in Biomass to Liquid Conversion ,Catalysts , Vol. 2 (2),pp 303326 , 2012, https://doi.org/https://doi.org/10.3390/catal2020303
, Biorefineries?: Achievements and challenges for a bio-based economy ,Front. Chem. , Vol. 10 ,pp 973417 , 2022, https://doi.org/https://doi.org/10.3389/fchem.2022.973417
, Pretreatment methods of lignocellulosic biomass for anaerobic digestion ,AMB Express , Vol. 7 ,pp 72 , 2017, https://doi.org/https://doi.org/10.1186/s13568-017-0375-4
, Evaluation of different pretreatment processes of lignocellulosic biomass for enhanced biomethane production ,Energy Fuels , Vol. 31 (10),pp 1033510347 , 2017, https://doi.org/https://doi.org/10.1021/acs.energyfuels.7b02045
, Different pretreatment technologies of lignocellulosic biomass for bioethanol production: An overview ,Energies , Vol. 199 ,pp 117457 , 2020, https://doi.org/https://doi.org/10.1016/j.energy.2020.117457
, Updates on the pretreatment of lignocellulosic feedstocks for bioenergy production a review ,Biomass Convers. Biorefinery , Vol. 8 ,pp 471483 , 2018, https://doi.org/https://doi.org/10.1007/s13399-017-0269-3
, Pretreatment of lignocellulosic wastes for biofuel production: A critical review ,Renew. Sustain. Energy Rev. , Vol. 90 ,pp 877891 , 2018, https://doi.org/https://doi.org/10.1016/j.rser.2018.03.111
, Pretreatment of wheat straw by white-rot fungi for enzymic saccharification of cellulose ,Eur. J. Appl. Microbiol. Biotechnol. , Vol. 18 ,pp 350357 , 1983, https://doi.org/https://doi.org/10.1007/BF00504744
, A viable technology to generate third-generation biofuel ,J. Chem. Technol. Biotechnol. , Vol. 86 (11),pp 13491353 , 2011, https://doi.org/https://doi.org/10.1002/jctb.2666
, Review of 12 Principles of Green Chemistry in Practice Review of 12 Principles of Green Chemistry in Practice ,Int. J. Sustain. Green Energy , Vol. 6 (3),pp 3948 , 2017, https://doi.org/https://doi.org/10.11648/j.ijrse.20170603.12
, Plant-based green synthesis of metallic nanoparticles?: scientific curiosity or a realistic alternative to chemical synthesis? ,Nanotechnol. Environ. Eng. , Vol. 4 (1), 2016, https://doi.org/https://doi.org/10.1007/s41204-016-0004-5
, Green Synthesis of Metallic Nanoparticles?: Applications and Limitations ,Catalysts , Vol. 11 (8),pp 902 , 2021, https://doi.org/https://doi.org/10.3390/catal11080902
, The green economy transition?: the challenges of technological change for sustainability ,Sustain. Earth Rev. , Vol. 3 (1), 2020, https://doi.org/https://doi.org/10.1186/s42055-020-00029-y
, Simultaneous production of biocrude oil and recovery of nutrients and metals from human feces via hydrothermal liquefaction ,Energy Convers. Manag. , Vol. 134 ,pp 340346 , 2017, https://doi.org/https://doi.org/10.1016/j.enconman.2016.12.052
, Plasma Gasification of Two Waste Streams: Municipal Solid Waste and Hazardous Waste from the Oil and Gas Industry ,Energy Procedia , Vol. 105 ,pp 41594166 , 2017, https://doi.org/https://doi.org/10.1016/j.egypro.2017.03.882
, Lignocellulosic biomass as sustainable feedstock and materials for power generation and energy storage ,J. Energy Chem. , Vol. 57 ,pp 247280 , 2021, https://doi.org/https://doi.org/10.1016/j.jechem.2020.08.060
, - https://advancedbiofuelsusa.info/global-energy-demand-to-grow-47-by-2050-with-oil-still-top-source-us-eia , 2021,
- https://www.eia.gov/todayinenergy/detail.php?id=49876 USA, 2021,
- ,
How Do Industrial Ecology, Energy Efficiency, and Waste Recycling Technology ( Circular Economy ) Fit into Chinas Plan to Protect the Environment? Up to Speed ,Recycling , Vol. 7 (6),pp 83 , 2022, https://doi.org/https://doi.org/10.3390/recycling7060083 - https://www.clientearth.org/latest/latest-updates/stories/fossil-fuels-and-climate-change-the-facts , 2022,
- https://www.un.org/en/chronicle/article/goal-7-ensure-access-affordable-reliable-sustainable-and-modern-energy-all , 2015,
- https://www.scientificamerican.com/article/does-burning-garbage-to-produce-energy-make-sense , 2011,
Bioeconomy: Markets, Implications, and Investment Opportunities ,Economies , Vol. 7 (3),pp 73 , 2019, https://doi.org/https://doi.org/10.3390/economies7030073
, Lignocellulosic Biomass?: Understanding Recalcitrance and Predicting Hydrolysis ,Front. Chem. , Vol. 7 ,pp 874 , 2019, https://doi.org/https://doi.org/10.3389/fchem.2019.00874
, - https://www.diva-portal.org/smash/get/diva2:10498/FULLTEXT01.pdf , 2006,
A concise review of current lignin production, applications, products and their environmental impact ,Ind. Crops Prod. , Vol. 139 ,pp 111526 , 2019, https://doi.org/https://doi.org/10.1016/j.indcrop.2019.111526
, Chemical and Physicochemical Pretreatment of Lignocellulosic Biomass: A Review ,Enzyme Res. , 2011, https://doi.org/https://doi.org/10.4061/2011/787532
, Magnetic nanoparticles?: preparation, physical properties, and applications in biomedicine ,Nanoscale Res. Lett. , Vol. 7 ,pp 144 , 2012, https://doi.org/https://doi.org/10.1186/1556-276X-7-144
,