The energy and water resources are strongly dependent on each other and are investigated through a joint method: the energy-water nexus. The first energy-water nexus definition was published by Gleick in 1994 [1]. He argues, that energy is needed for water - for moving water, for groundwater pumping and desalination - and that in turn water is needed for energy - for the cooling process of thermal power plants and for the mining and processing of primary energy sources. One example of water for energy given is that a coal-fired power plant with a once-through cooling system uses 10 times the amount of water as of coal in terms of their weight.
With the transition of the energy system, the water system will also change and the opportunity to build a fair energy water management for both systems could be seized. For example, around one half of the total water extraction in Germany is used for cooling purposes in the energy sector for mostly fossil power plants [2] and fossil power plants will phase out with the energy transition. With the increasing usage of bioenergy and the massive cultivation of energy crops (e.g., rape seed, starchy crops and woody crops) the scarcity of land area is increasing. One way to increase the yield of the energy crops is to irrigate them, which could lead to a new water demand for the energy sector. How the energy sector evolves is highly dependent on the national climate target and since energy and water resource systems are strongly interlinked, it is assumed that the changes in the water sector will relate directly to the national climate target.
The concept of the nexus was promoted in the late 2000s in conferences such as the Bonn Nexus Conference [3]. The first papers addressing the nexus were conceptual, for example, Bazilian et al. explain the interactions between the resource systems and prove them with statistical values [4]. Leck et al. review on the one hand scientific literature of the theoretical nexus approach and on the other hand the political dimensions of how nexus ideas find their way into the political discussion [5]. They conclude that researchers have to overcome inter disciplinary barriers and stakeholder institutional barriers, but the nexus concept could be applied in practice with a stronger cooperation. One example where this has been applied in practice is the cooperation between the World Water Council and the World Energy Council, who support the water-energy nexus topic with jointly organized conferences and research activities [6]. The nexus concept was used in research to conduct quantitative case studies investigating the current and past energy-water interactions for different regions. For example, Siddiqi and Anadon [7] compared the current water for energy and energy for water interactions for countries in the Middle East and North Africa (MENA) region and identified countries with extremely high energy demands for water abstraction and desalination. For a European case study Larsen and Drews [8] set the focus on how to quantify the water use for electricity generation. The energy water nexus was also analysed in case studies of individual countries like China [9] by visualising the water utilisation with a Sankey diagram. Another example of analysing the nexus interactions was conducted through a Mexican case study [10] where the interactions for past data years are quantified and possible risks were identified but without modelling future scenarios. The commonality of these four studies is that they didn’t develop a methodology to calculate future nexus interactions under different scenario assumptions. D’Odorico et al. review existing literature to examine the essential parts of the energy-water-food nexus at a global scale and assert that water availability is a crucial constraining factor for future energy and food supply [11]. With regard to the local water availability of Germany we put together the results of the water demand from the energy sector with public and industrial water demands and compare it with historic data of water availability.
An overview of methodologies for nexus assessments for a wide variety of research questions in the field of the water-energy-food nexus was developed by Albrecht et. al [12]. They pointed out that "only 30% of peer-reviewed literature reviewed (73 of 245 articles) present nexus methods” [12] and a small share of those methodological papers present integrated scenario analysis. When it comes to modelling the energy and water system conjointly for future scenario analysis there are two methods: the first is to use one model for each resource system and couple them together. An example is CLEWS (climate, land (food), energy & water strategies) [13], where energy, water and land use models are linked. Here, three main findings were identified: electricity demand increases when water demand increases, more energy intensive methods will be used to treat the water (desalination) and less energy can be produced from hydropower due to lower river water levels resulting in a change of the power system. These nexus aspects, however, are not relevant for a German case study, because a change in water treatment technologies is not likely to happen and the amount of hydropower potential for electricity generation is negligible. Depending on the case study, sometimes only two of their models are coupled, for example in the case study of Ethiopia’s lake Tana sub-basin Karlberg et. al use the energy model LEAP and the water model WEAP to address the cross-sectoral interactions and competitive resource uses [14]. Another assessment with the model coupling method was conducted by Flörke [15] with the water resource model WaterGap and inputs from climate, socio-economic and electricity models with a good representation of cooling water demand and water availability. Mouratiadou et al. analysed besides the cooling water demand also irrigation water demands in a global water demand study with the modelling framework LPJmJ-MAgPIE-REMIND which are a hydrological, a land-use and an energy system model coupled together [16]. They deduce that irrigation of energy crops is the most influential factor for future water demand. In order to deepen the understanding of the circumstances under which this biomass irrigation process will be applied in future, we present a new methodology in this paper, which integrates the investment decision for irrigation into an energy system model.
The second method to model nexus interactions is to integrate the water and energy system into one model. There are a variety of model types addressing the energy-water nexus, e.g., electricity-water models, integrated assessment models (IAM), newly developed nexus models or energy system models. Faeth et al. built an electricity-water model, where the modelled cooling water demand results in less investment choices in nuclear power systems: a CO2-free technology with a high consumption of cooling water [17]. Also Fricko et al. investigate the global cooling water demand and its resulting thermal pollution with the MESSAGE IAM framework and argue that nuclear power plants and Carbon Capture and Storage (CCS) power plants could contribute to localized water stress and thermal pollution [18]. The integrated assessment model GCAM was extended with a detailed water module which can analyse future water demand for different sectors in a static manner for future scenarios [19]. Hejazi et al. conclude that water prices on crop choices should be further investigated which will be analysed in this paper for bioenergy crops. Another research focusing mainly on agricultural water was set with the integrated nexus model AWEFSM, which was developed recently from Li et al. [20] to investigate trade-offs in limited water-energy-food resource systems. Also Engström et al. expand the nexus concept by quantifying the direct and indirect water uses of their energy system model LEAP and showing that local stakeholders can influence the water systems in national and global levels [21].
The integrated modelling of the water system in an energy system model was performed for three TIMES-based energy system models. Dubreuil et al. [22] incorporate a water module in their global model TIAM-FR and focuses on the energy demand for water treatment and irrigation water demands. The other two studies are reports from the World Bank and are quite similar from a methodological point of view: the focus is set on incorporating the cooling water demand and its pricing. This was done for a South African case study with the model SATIM-W [23]. Further, a Chinese case study was conducted with the model TIMES-ChinaW [24]. So far there was no analysis of the water-energy nexus with an energy system model addressing the cooling and irrigation water demands at the same time and using the optimization framework for energy and irrigation technology investment decision support. For this case study of Germany, cooling water demand as well as irrigation water demand for energy crops are important aspects of the future energy-water nexus and are analysed simultaneously and integrated with the total water demand and availability. The analysis is structured as follows:
-
Water demand for cooling technologies: Thermal power stations are highly dependent on cooling water mostly taken from rivers. Due to greenhouse gas mitigation strategies and the decided nuclear power phase-out, the number of fossil power stations will strongly decrease in the future. This change might have the highest impact on overall water projections. Since the national energy policies will affect the cooling water demand so significantly, an ex-post analysis of the water demand of an energy scenario analysis is an adequate method.
-
Irrigation of energy crops: Irrigation will become more important not only due to climate warming but because it could be an option to increase the yield of energy and food crops. Therefore, the optimal amount of irrigation could enhance the land productivity, which is an appealing option for countries with land scarcity. Since investment decisions are necessary for building the irrigation infrastructure to take advantage of the increased biomass potential, this interaction was integrated in the energy system model.
-
Total water demand: To get an overall picture of the total future water resource use, the public and industrial water supply and its outlook was considered based on a literature assessment.
-
Total renewable water supply: A regression analysis based on the last 25 years of renewable water supply was conducted in order to estimate the future limits of a water-stress free resource use.
For this study an integrated assessment approach with the energy system model TIMES PanEU was chosen where either the model or results analysis were adjusted to incorporate the nexus aspects mentioned in the previous section. In the following sections the methodology to incorporate each nexus aspect is described in detail:
The projection of the future water demand requires a scenario analysis of the future energy system. Since different cooling technologies need different amounts of water, a detailed assessment of the different cooling technologies of the German power generation system is necessary. Official data are not available, but Larsen and Drews [8] gathered information about the biggest European power plants and came up with an approximate overview of the cooling technology distribution for each country. This work is a good starting point for further European analyses; however, it does not distinguish between the power generation plant types (electricity plants and combined heat and power (CHP) plants). To come up with a more precise picture, the distribution of the cooling technologies was analysed by a literature review of the 200 biggest power plants in Germany. Their capacity, power generation per year, mode of operation (with or without CHP) and exact location are easily accessible. However, the type of cooling technology of the power plants is rarely available. When it was not possible to find such information of a certain power plant, a remote sensing analysis of these plants was executed with Google Maps. The gathered data set adds up to the distribution of the cooling technologies (Figure 1), divided into tower cooling, once trough cooling and hybrid cooling:
Distribution of cooling technologies of the German power sector
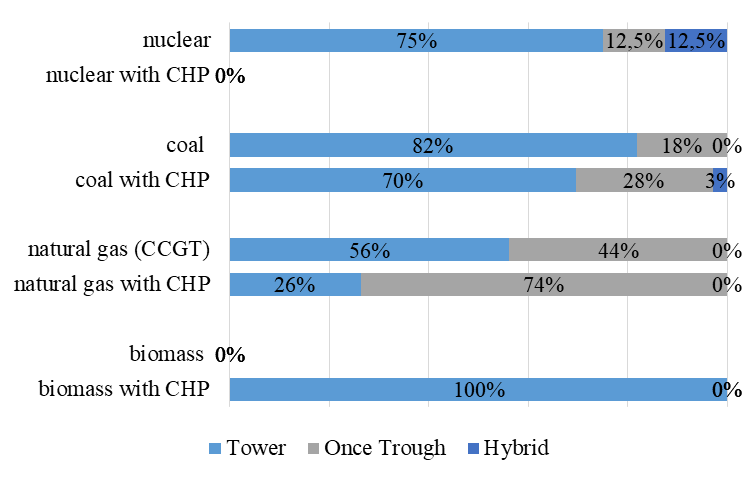
The CHP plants do not need cooling in winter months, but since there is no district heat demand in summer, they need water cooling. This is mostly done by once trough cooling, which requires high water withdrawals.
The second part of the future water use assessment of the energy system are the factors for the water use and consumption per TWh of electricity produced. This data is taken from a comparative US study by Macknick [25]. Larsen and Drews [8] also analysed these factors extensively, but aggregated the types of power plants too much so that including them in the TIMES energy system model is not suitable. Macknick [25] calculates median factors for water use of different energy carriers, plant types and cooling technologies at the same level of detail as the energy system model of real-life observed water uses. However, the compared water uses still result in a wide spread range of water factors, hence, calculating with the median value contains these uncertainties. Moreover, further uncertainties might occur by applying the US dataset to Germany due to different climatic conditions in both regions.
By coupling the cooling technologies distribution, the factors of water use and the energy system model, future water analyses can be conducted. With the energy system model TIMES Pan EU different policy scenarios were calculated as a basis for the further processing. TIMES is an economic model generator which is used with TIMES PanEU for German and European applications. The model provides a technology-rich basis for representing energy dynamics over a time horizon from 2010 to 2050. It is used for the analysis and optimisation of the entire energy sector which is driven by the end use energy service demands (e.g. train transport, residential lighting, high temperature heat requirement in the steel industry, etc.) for each region. Moreover, estimates of the existing stocks of power plants, transportation means, industrial plants, and the features of available future technologies are stored in the database, in addition to present and future sources of primary energy supply and their potentials [26]. The potentials of renewable energies for Germany is given in the model based on [27] for solar power and [28] for wind power. The biomass potential relies on the available land area for cultivation according to [29]. This land area can be used for the cultivation of rape seed, starchy crops, sugar crops and woody crops. The energy crops can be converted to various bioenergy carriers e.g. biodiesel or biogas, which can be used in the entire energy sector. Finally, the model optimises the overall system cost to supply the required energy services and provides the investment and operation decisions as well as the energy trade within the model regions.
The simulation of the present cooling water use and cooling water consumption of the energy sector resulted in slightly different values than the ones obtained from statistics due to the uncertainties mentioned above. The most probable explanation is, that the share of once through cooling technologies is underestimated because power plants often operate with two cooling technologies and combine their tower cooling system with an additional once trough river cooling. In order to calibrate the water simulation to the actual statistics a correction factor was applied.
Irrigation as an investment option is a good application of the integration into the energy optimisation model. The yield increase of biomass when irrigation is applied was derived from MAgPIE, (Model of Agricultural Production and its Impact on the Environment) which is a land use optimisation model [30]. This benefit faces the trade-off with the costs of building the irrigation infrastructure. Resulting from previous TIMES-PanEU runs, the optimal biomass cultivation in Germany would be woody and starchy crops from 2030 on. For that reason, drip irrigation was chosen as irrigation technology and the corresponding costs were applied [31]. Additionally, the water pricing, which varies depending on many factors, should be considered. The first factor is the provincial governmental water pricing which ranges from 0 – 0.12 EUR/m³ depending on the region within Germany. Then, there are eventually costs for building an abstraction structure for either ground water or surface water when there is no current water use. Another option is to obtain water from irrigation water associations with an average price of 0.20 EUR/m³ [31]. In order to see the differences of irrigation uses in the model, two extreme scenarios were built to demonstrate the range of possible irrigation water uses. The first scenario with low irrigation costs contains just the costs of the irrigation infrastructure (it was assumed that a given water abstraction structure can be used and the regional government does not charge for irrigation water use). The second scenario with high irrigation costs contains both the costs for the irrigation infrastructure and the costs of an irrigation water association.
In order to get an overall picture of the future water use and consumption, a prediction about population growth [32] and gross value added [33] was implemented and correlated with the public water supply and the industrial water supply. The water withdrawal numbers for the period from 2010 [34] and 2015 [2] were gathered from statistical values. For the future time period, it was assumed that the public water supply fully correlates with the population trend as well as that the industrial water demand fully correlates with the predicted gross value added for the different industry branches. The municipal water demand per capita remained constant over the last 13 years in Germany [35], so that a full correlation with the population trend can be applied. The industrial water demand is divided into eight branches (e.g. chemical industry, food and tobacco, pulp and paper) and the specific gross value added for the industry branches were applied as a correlation to the industrial water demand development.
According to literature [35], the long term average value of water availability of renewable water resources in Germany adds up to 188 billion m³ per year, which is a value calculated for the time period of 1961-1990. For this paper, a linear regression analysis with the actual time period of 1990 - 2017 is conducted [36].
Three energy scenarios (Table 1) were calculated to analyse possible development in the energy system depending on political regulation for Germany.
Energy scenario description
Scenario name |
Scenario description |
|
German target |
EU target |
|
ETS GHG90 |
ETS, CO2 Cap 90% GHG reduction target 95% cumulative GHG reduction target |
ETS, CO2 Cap 80% GHG reduction target 80% cumulative GHG reduction target |
CUM95 |
The first scenario is an EU-ETS Scenario, where a CO2 Cap is applied for the European energy sector and the energy intensive industry branches. The second scenario includes a German national 90% greenhouse gas (GHG) reduction target and an EU 80% GHG reduction target, which is applied over all sectors. In this scenario, two different price assumptions for the irrigation water were made to show the demand response. The third scenario is a cumulative GHG cap over the years 2025 -2050, which corresponds to a 95% GHG mitigation scenario for Germany and to an 80% mitigation scenario for Europe.
The cooling water demand decreases accordingly with the energy transition goal (Figure 2). The more ambitious the GHG reduction target, the further decline can be observed in the cooling water demand. This correlation is obvious because electricity generation by wind power plants or by photovoltaic (PV) plants do not require water for operation (the minimal water demand for cleaning the PV panels during maintenance was neglected). In the scenario with a cumulative GHG bound, action to reach this target is applied much earlier than in the other scenarios, which results in a stronger decline of cooling water use.
Water use for cooling technologies and lignite mining
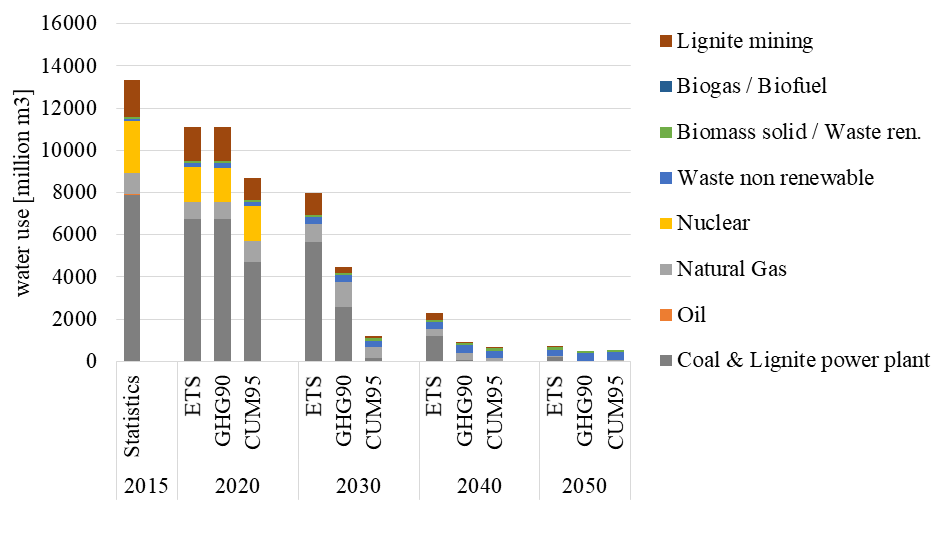
In every scenario there is a strong decline from 13 billion m³ of water use in 2015 to approximate 0.4- 0.9 billion m³ of water monitored which corresponds to a reduction of 93-97%. In the energy scenario analyses, the option of any CCS technology was excluded from the possible GHG mitigation measures, because this technology bears a lot of acceptance problems in the society. If any CCS technologies would be applied in the future, there would be an additional water demand that is not taken into account in this study.
The cooling water is mainly taken from rivers and, after the cooling process, the majority of the withdrawn water is fed back into the river. This process causes a temperature increase of the river water. The largest river in Germany, the Rhine, is currently the most heat polluted river basin of the world according to Raptis et al. [37], but this situation will change as soon as the stricter climate target is set for the energy system.
The share of the irrigated land area for biomass cultivation is shown in this section. The biggest yield increase when irrigation is applied can be accomplished for woody biomass. For the other types of biomass, it isn’t cost-effective to build the irrigation infrastructure because those crops already have almost nearly optimal soil humidity conditions and their yield could be increased just slightly. Therefore, when including the specific yield increase of the biomass species and the costs for the irrigation infrastructure, the TIMES PanEU model decides to invest in the irrigation of woody crops only (Figure 3). At the same time, woody crops are the favourite bioenergy crops after the change from 1st generation biofuels to 2nd generation biofuels around 2030. From the analysis of different climate policies, it can be shown that the higher the GHG mitigation goal is, the more biomass is used in the energy system and the more irrigated woody biomass cultivation is used.
Land use for energy crops (rainfed and irrigated)
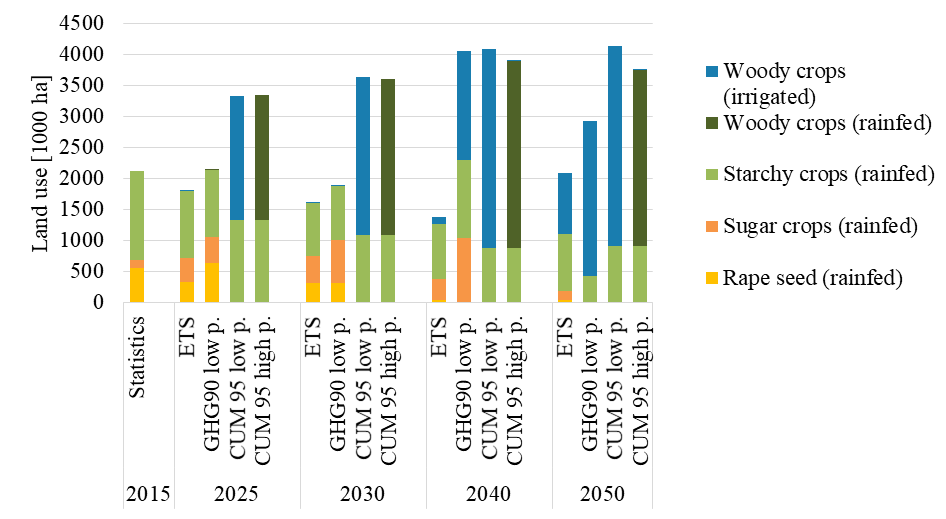
This trend is contradictive to the results of the cooling water demand and a trade-off relation between future water use and high GHG reduction can be observed for strong climate goals. In the scenario CUM95, a 95% cumulative greenhouse mitigation between the years 2025 and 2050 is modelled, which results in a strong increase of biomass use from 2025 on. With this CUM95 climate goal scenario, the effect of different water pricing was analysed. When high irrigation prices of additional 0.20 EUR/m³ of water are implemented into the energy system model, the model chooses the rainfed woody crop cultivation option only. This occurs because the irrigation option to increase the yield is not cost-effective anymore. This result proves that the pricing of irrigation water would be an actual tool for regional governments to regulate the irrigation practice within their region.
The current water demand for irrigation in Germany is supplied with groundwater and if the option of recycling water is not considered, a trend will emerge with significant groundwater usage in the future.
Whereas the population forecast is decreasing, the predicted gross value added is increasing for most industry branches, which leads to an overall water demand increase. All the industry branches were summed up to one water use value for a clearer arrangement and added to the previously discussed water uses in Figure 4:
Total water use
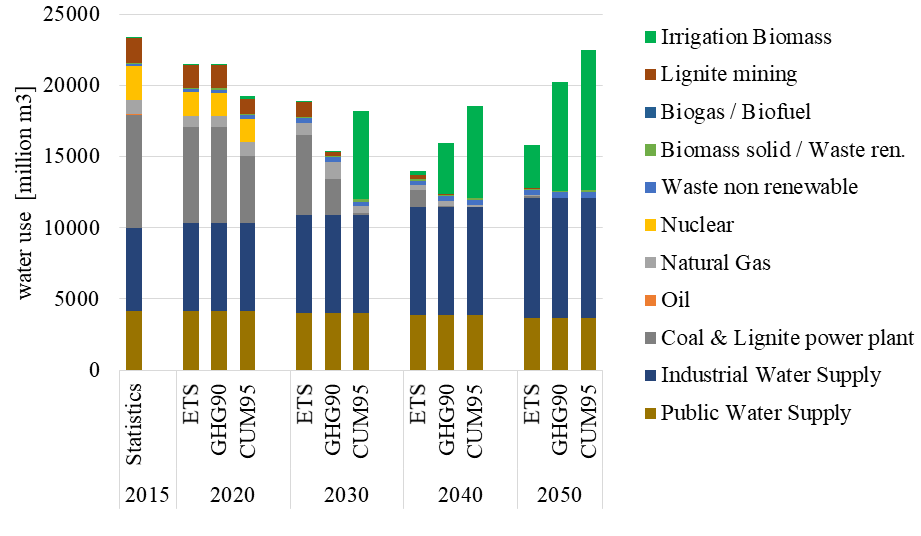
The industry water uses and the public water supply are increasing from 10 billion m³ of water use in 2015 to 12 billion m³ in 2050, which also partly changes the overall picture. The industrial water demand is mainly supplied through river water (72%) [34] and the public water supply mainly through groundwater (78%) [38].
In total, the water use declines from 23 billion m³ in 2015 to 15.6-22.5 billion m³ water use in 2050, depending on the scenario assumptions. This corresponds to a decline of 4-32%. Overall, river water is currently the main water withdrawal source in Germany supplying approximately 80% of demand. If future irrigation water is fully supplied by groundwater, groundwater will become the main future water source with 60 to 70% of total water withdrawal depending on the scenario.
In the time period of 1990 - 2017, the renewable water resource averaged at 175 billion m³ per year, and the regression analysis shows a slight decrease of 0.5% yearly (Figure 5):
Renewable water resource with linear regression line in Germany
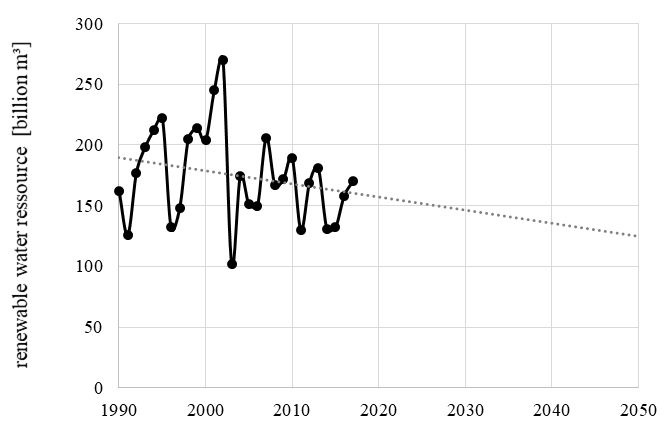
The calculated annual water availability in 2050 is 131 billion m³ of water, but since the standard deviation of the past data points is as high as 39 billion m³, the regression line needs to be considered as the long term average and the actual value in 2050 could vary between 92 and 170 billion m³. An environmentally friendly water use without water stress implies the usage of less than 20% of the available water, which has varied between 54 and 20 billion m³ of water in the past. For the future data point in 2050, the optimal water use should be less than 26 billion m³ based on the average value or less than 18-34 billion m³ of water when the standard deviation is taken into account.
It could be that this regression analysis with the past 27 data years of the renewable water resource and its extrapolation to the future is a too pessimistic guess, since the period with lower than usual water availability from 2003-2017 could be just temporal fluctuations from the long term average value. Nevertheless, this assessment can be seen as a worst case scenario for the future water availability, which is most valuable for energy-water nexus analyses.
Climate change projections differ greatly for the different regions within Germany – for example the groundwater regeneration in the German state of Hessen is projected to increase by 25% until 2050, while in the state of Brandenburg the groundwater regeneration is projected to decrease by 40% [38]. These regional extremes might even out and disappear when a national average is applied.
The total future water demand in 2050 in Germany varies according to different climate policy scenarios between 15.6 and 22.5 billion m³ of water. When the total water demand is compared to the regression analysis of the past renewable water availabilities, the risk of future water stress can be calculated to a probability of 30% for the highest climate target, if the standard deviation of past data points is taken into account. This assessment is based on a German national scale and could be different for the regions within Germany, when a finer regional scale would be applied. According to literature [38], Brandenburg faces the strongest climate change induced decrease of groundwater availability, and measures should be taken to support the regeneration of groundwater. The future possibility of irrigating biomass plants to increase the yield should be avoided in this region by increasing the price for groundwater for irrigation in Brandenburg. Scenario analysis has shown that water pricing would incentivise the regulation of the irrigation practice appropriately. Moreover, the groundwater quality and quantity in Brandenburg is currently strongly affected by the biggest lignite surface mining field of Germany [39]. In order to protect the water resource, a coal exit strategy for power generation should be implemented as fast as possible.
The total water demand changes from mostly river water withdrawals for cooling purposes to more groundwater water consumption for irrigation purposes. For the river ecosystems, this is a beneficial development, because heat pollution can be avoided and temperature sensitive species can be protected. The Rhine is currently the most thermally polluted river of the world and regional governments could help to improve the water quality by setting an adequate price for the water withdrawal for cooling purposes. In many regions within Germany the water price for cooling purposes amounts to around 90% less than the water price for public water uses.
It might be that the groundwater regeneration partially decreases in the future, due to the fact that woody crop cultivation consumes more water than regular agricultural crops. The amount of projected irrigation water for biomass is dependent on the use of biomass in the future energy system. The expansion of biomass use is furthermore dependent on other renewable energy developments like wind and solar power. If the assumed maximum deployment of wind and solar power could increase due to technological progress and innovative technologies (e.g., agricultural PV or floating PV), an energy system with less biomass use and correspondingly less irrigation water demand could be realised. On the other hand, the biomass use and the irrigation water demand could be even higher than the scenario results if biomass CCS technologies would be employed in future.
With the results of a massive increase of future irrigation water demand for woody crops, it should be a next step to think of alternative ways of water supply, mainly how recycled waste water could be applied for irrigation. From a quantitative perspective, there are 9.6 billion m³ of treated waste water discharged every year in Germany and 7.6 billion m³ of predicted irrigation water demand, which could be totally supplied by recycled water. With this measure, the risk of future water stress could be nearly eliminated. From a qualitative perspective, the waste water treatment standards are quite high and 90% of the waste water plants feature denitrification and phosphorus removal treatment steps in addition to the mechanical and biological standard treatment. There are already some research pilot projects which apply treated waste water irrigation for short rotation forestry [40], but the legal basis is not defined yet and could vary between local governments.
Overall, the energy transition can have a significant impact on the water use. More specifically, the demand of cooling water will decrease drastically whereas the demand of irrigation water and industrial water will increase according to this scenario analysis. There is a small risk of future water stress that can be eliminated by the use of recycled water for irrigation.
The research presented in this contribution is sponsored by the German Federal Ministry of Education and Research (Reference: 03SFK4M1, Consortium ENavi, Kopernikus). We would like to thank Miodrag Stevanovic who provided the values of the biomass yield increase by MagPIE and two anonymous reviewers of this paper and the editors.
AWEFSM - Agricultural Water-Energy-Food Sustainable Management
CCS - Carbon capture and storage
CHP - Combined heat and power
CLEWS - Climate, land (food), energy & water strategies
GCAM - Global change assessment model
GHG - Greenhouse gas
IAM - Integrated assessment model
LEAP - Long range Energy Alternatives Planning System
LPJmL - Lund-Potsdam-Jena managed Land
MAgPIE - Model of Agricultural Production and its Impact on the Environment
MENA - Middle East and North AfricaPhotovoltaic
REMIND - Regionalized Model of Investments and Development
TIMES - The Integrated MARKAL-EFOM System
WEAP - Water Evaluation And Planning System
Water and Energy ,Annu. Rev. Energy Environ. ,pp 267-299 , 1994
, - Nichtöffentliche Wasserversorgung und nichtöffentliche Abwasserentsorgung , Fachserie , 2016
Understanding the Nexus ,Background Paper for the Bonn2011 Conference: The Water, Energy and Food Security Nexus. , (November),pp 1-52 , 2011
, Considering the energy, water and food nexus: Towards an integrated modelling approach ,Energy Policy , Vol. 39 (12),pp 7896-7906 , 2011, https://doi.org/10.1016/j.enpol.2011.09.039
, Tracing the Water–Energy–Food Nexus: Description, Theory and Practice ,Geography Compass , Vol. 9 (8),pp 445-460 , 2015, https://doi.org/10.1111/gec3.12222
, - WWC and WEC team up to highlight the water-energy nexus, 2014, https://www.worldwatercouncil.org/en/wwc-and-wec-team-highlight-water-energy-nexus, [Accessed: 8.5.2020]
The water-energy nexus in Middle East and North Africa ,Energy Policy , Vol. 39 (8),pp 4529-4540 , 2011, https://doi.org/10.1016/j.enpol.2011.04.023
, Water use in electricity generation for water-energy nexus analyses: The European case ,Science of the Total Environment , Vol. 651 ,pp 2044-2058 , 2019, https://doi.org/10.1016/j.scitotenv.2018.10.045
, China's energy-water nexus in 2009 by Sankey diagram ,Chemical Engineering Transactions , Vol. 42 (2010),pp 31-36 , 2014, https://doi.org/10.3303/CET1442006
, Energy and water linkage in Mexico ,6th Dubrovnik Conference on Sustainable Development of Energy, Water and Evironment Systems , Vol. 4 (1),pp 15-22 , 2011, https://doi.org/10.5383/swes.04.01.002
, The Global Food-Energy-Water Nexus ,Reviews of Geophysics , Vol. 56 (3),pp 456-531 , 2018, https://doi.org/10.1029/2017RG000591
, The Water-Energy-Food Nexus : A systematic review of methods for nexus assessment The Water-Energy-Food Nexus : A systematic review of methods for nexus assessment , 2018, https://doi.org/https://doi.org/10.1088/1748-9326/aaa9c6
, Adding value with CLEWS - Modelling the energy system and its interdependencies for Mauritius ,Applied Energy , Vol. 113 (January),pp 1434-1445 , 2014, https://doi.org/10.1016/j.apenergy.2013.08.083
, Tackling Complexity : Understanding the Food-Energy- Environment Nexus in Ethiopia ’ s Lake Tana Sub -basin ,Water Alternatives , Vol. 8 (1),pp 710-734 , 2015
, Future changes of freshwater needs in European power plants ,Management of Environmental Quality: An International Journal , Vol. 22 (1),pp 89-104 , 2011, https://doi.org/10.1108/14777831111098507
, The impact of climate change mitigation on water demand for energy and food: An integrated analysis based on the Shared Socioeconomic Pathways ,Environmental Science and Policy , Vol. 64 ,pp 48-58 , 2016, https://doi.org/10.1016/j.envsci.2016.06.007
, A clash of competing necessities ,CNA Analysis , (July), 2014
, Energy sector water use implications of a 2°C climate policy ,Environmental Research Letters , Vol. 11 (3), 2016, https://doi.org/10.1088/1748-9326/11/3/034011
, Long-term global water projections using six socioeconomic scenarios in an integrated assessment modeling framework ,Technological Forecasting and Social Change , Vol. 81 (1),pp 205-226 , 2014, https://doi.org/10.1016/j.techfore.2013.05.006
, An optimal modelling approach for managing agricultural water-energy-food nexus under uncertainty ,Science of the Total Environment , Vol. Volume 651 ,pp 1416-1434 , 2019, https://doi.org/https://doi.org/10.1016/j.scitotenv.2018.09.291
, Cross-scalewater and land impacts of local climate and energy policy-A local Swedish analysis of selected SDG interactions ,Sustainability (Switzerland) , Vol. 11 (7), 2019, https://doi.org/10.3390/su11071847
, Water modeling in an energy optimization framework - The water-scarce middle east context ,Applied Energy , Vol. 101 ,pp 268-279 , 2013, https://doi.org/10.1016/j.apenergy.2012.06.032
, Modeling the Water-Energy Nexus: How Do Water Constraints Affect Energy Planning in south Africa? , 2017
, Thirsty Energy: Modeling the Water-Energy Nexus in China , 2018
, Operational water consumption and withdrawal factors for electricity generating technologies: A review of existing literature ,Environmental Research Letters , Vol. 7 (4), 2012, https://doi.org/10.1088/1748-9326/7/4/045802
, Documentation for the TIMES Model PART I - Concepts and Theory , (July),pp 151 , 2016
, Räumlich differenzierte Flächenpotentiale für erneuerbare Energien in Deutschland ,BMVI-Online-Publikation , (08), 2015
, - Langfristszenarien und Strategien für den Ausbau der erneuerbaren Energien in Deutschland bei Berücksichtigung der Entwicklung in Europa und global „Leitstudie 2010“ , 2010
- Biomasse-Potentiale, 2016, https://bioenergie.fnr.de/bioenergie/biomasse/biomasse-potenziale/, [Accessed: 6.3.2019]
Global food demand, productivity growth, and the scarcity of land and water resources: A spatially explicit mathematical programming approach ,Agricultural Economics , Vol. 39 (3),pp 325-338 , 2008, https://doi.org/10.1111/j.1574-0862.2008.00336.x
, Investitionen und Verfahrenskosten für die Feldbewässerung ,Landtechnik , Vol. 65 ,pp 189-193 , 2010
, Bevölkerung und Erwerbstätigkeit - Bevölkerungsfortschreibung auf Grundlage des Zensus 2011 , Vol. Fachserie , 2016
, Klimapfade für Deutschland ,pp 286 , 2018
, - Nichtöffentliche Wasserversorgung und nichtöffentliche Abwasserentsorgung , Fachserie , 2010
- Wasserressourcen und ihre Nutzung, , https://www.umweltbundesamt.de/daten/wasser/wasserressourcen-ihre-nutzung#textpart-1, [Accessed: 15.2.2019]
- , Mitteilung vom 15.04.2019, 2019
Global thermal pollution of rivers from thermoelectric power plants ,IOP Publishing , 2016, https://doi.org/10.1088/1748-9326/11/10/104011
, Grundwasser in Deutschland , 2008
, Daten und Fakten zu Braun- und Steinkohlen , 2017
, - Bewässerung mit Abwasser Regionale Ressourcennutzung aus eigener Kraft ,