The increase in the demand for fresh water is a worldwide issue, and a large portion of the available freshwater bodies are under constant contamination. Wastewater effluents are a major source of micropollutants in the water cycle, and these pollutants eventually find their way to drinking-water supplies like rivers, lakes, or groundwater aquifers [1], [2]. Several environmental contaminants, including, but not limited to, endocrine disrupting compounds (EDCs), and specifically pharmaceutically active compounds (PhACs), have been detected globally and are a cause for concern due to their adverse effects on the environment and on public health [1]. Polar contaminants like pharmaceuticals and pesticides have been found to leach into ground waters and adsorb on aquifers, thus they can appear in drinking water with many of these contaminants present simultaneously [3]. Long term exposure to pharmaceuticals, even at trace levels, have been proven to have caused hormonal disruptions in fish and the decline of bird populations in some parts of the world [4], [5]. There is also a potential risk that the increase and accumulation of pharmaceuticals in the environment could pose a serious threat to human health in future [1].
Conventional water and wastewater treatment systems can partially remove some pharmaceuticals, however most compounds are not removed and are consequently detected in the outlet streams [6]. These systems generally use activated sludge to treat wastewater according to environmental standards, and many pharmaceuticals have hydrophilic characters, which limits their sorption to sludge [7], [8]. Non-conventional systems like membrane separation processes (MSP) could be used to compliment conventional systems and effectively remove PhACs from sewage and drinking-water [1]. In particular the water-scarce Middle East and North Africa region has suffered from seawater intrusion into aquifers, and has relied heavily on desalination technologies including MSPs to satisfy their water demand [9]. It is worthwhile then to look into the efficiency of these processes in the removal of contaminants and the different factors that are in effect.
MSPs are physical separation processes which are characterized by their high efficiency when removing contaminants, such as salt, heavy metals, and organic matter, and have shown good overall rejection values for pharmaceuticals [10]. These MSPs include processes such as forward osmosis (FO), membrane bioreactors (MBR), microfiltration (MF), ultrafiltration (UF), nanofiltration (NF), and reverse osmosis (RO) [11]. The classification of NF membranes lies in an area between UF and RO membranes, in fact tight NF membranes are similar to RO membranes, whereas a loose NF membrane are similar to UF membrane [12]. These membranes are typically advantageous in their lower operating pressure of 5-10 bar for brackish water, compared to 20+ bar in RO, in addition to the combination of high rejection for multivalent ions (>99%), and moderate rejections for monovalent ions (0-70%) [12]. Specifically the water-scarce Middle East and North Africa region has suffered from
NF membranes are known to be a reliable technology for water reuse, especially in processes where the complete removal of ions is not necessary [13]. The performance of NF membranes in rejecting PhACs has varied between different types of PhAC ranging from rejections of 0% to greater than 90% [11]. Radjenovic et al. performed experiments on twelve different pharmaceutical products. Nine out of twelve of the pharmaceuticals resulted in rejection rates greater than 95% and the other three were reported to have only slight rejections (~50%) [14]. The lower rejections were concluded to be due to the hydrophilic behaviors and the low molecular weights of the component. In another case, 52 different EDCs and pharmaceuticals, and their retention via NF were studied by Yoon et al. in order to gather certain patterns, and noted that hydrophobicity is a very important factor [15]. Even though there are still some controversial results when NF is concerned, it is agreed upon that tighter NF membranes are more efficient than looser membranes, reaching >99% of efficiency, yet the tighter membranes, suffer from a greater flux decline [11]. Sadmani et al. investigated the effect of natural water colloids and cations on the removal of PhACs using NF, and found that cations did not have a significant effect on rejection whereas colloidal matter resulted in a decrease of neutral compound rejection yet had no effect on charged compounds [16]. The influence of water matrices on the nanofiltration of PhACs was also investigated by Azais et al. through studying the effect of foulants such as humic acid and polysaccharides; membrane fouling caused by organic matter resulted in permeate flux decline throughout the process and can either increase or decrease PhAC rejection by adsorption on macromolecules or forming a fouling layer respectively [13].
Though most of the above-mentioned studies have focused on the removal of low concentrations (ng/L) of pharmaceuticals as occurring in the environment, another approach could tackle the issue from the source. In fact, based on a review on the pollution from drug manufacturing, pharmaceutical concentrations in the mg/L range are typically being detected in industrial effluents all around the world [17]. Some cities with pharmaceutical manufacturing facilities have been detecting high concentration in their receiving water bodies, making industrial waste streams a major contributor to the issue [18]. Hence, to potentially consider nanofiltration for the treatment of waste streams, tests need to be done at these relatively high concentration levels. Actually, De Souza et al. aimed to assess the performance of nanofiltration for the removal of an antibiotic in simulated pharmaceutical wastewater and used concentrations of 5, 25, and 50 mg/L of the pharmaceutical in solution [19]. Speculations on the mechanisms and the effect of pH were reported and NF was found to be viable in removing norfloxacin from effluents even at these high concentrations. In addition, even though nanofiltration has been shown to be a viable method for removing trace pharmaceuticals, information is still lacking when it comes to high concentrations of these emerging contaminants [20].
Moreover, monovalent salts have not been investigated extensively, and pharmaceutical industry waste streams have been known to be high in salts especially in sodium chloride. As an example, a study on pharmaceutical waste streams in Singapore found the TDS of the solution to be in the 20,000 mg/L range, with Sodium (Na+) and chloride (Cl-) concentrations responsible for more than half that value [21]. The effect of different salts such as sodium chloride (NaCl) calcium chloride (CaCl2) and sodium sulfate (Na2SO4) on the removal of Ibuprofen by NF membranes, and the mechanisms by which these salts affected the process was studied recently and found to be dependent on the type of salt used; the study did not however consider different types of pharmaceuticals, especially ones with no charge in solution [22].
This paper aims to study the removal of three different pharmaceuticals by a nanofiltration membrane in a concentration mode of filtration. Moreover, the effects of the monovalent salt sodium chloride (NaCl), at different concentrations, as well as the temperature on the removal of these pharmaceuticals are studied.
A bench-scale cross-flow membrane filtration system was used to perform all experiments in this study. The system uses a Hydracell M-03 positive displacement diaphragm pump (Wanner Engineering Inc., USA) to deliver feed water from a 25-Liter polypropylene tank to the filtration unit. The commercial membrane cell CF-042D (Sterlitech Corporation, USA) can hold a membrane coupon with an active area of 42 cm2. Pressure gauges and an in-line temperature sensor were used to constantly monitor the operating conditions. A food grade heat exchanger was used to cool the feed and maintain a constant temperature. A schematic flow diagram of the bench-scale membrane system is given in Figure 1.
Schematic flow diagram of the bench-scale membrane system
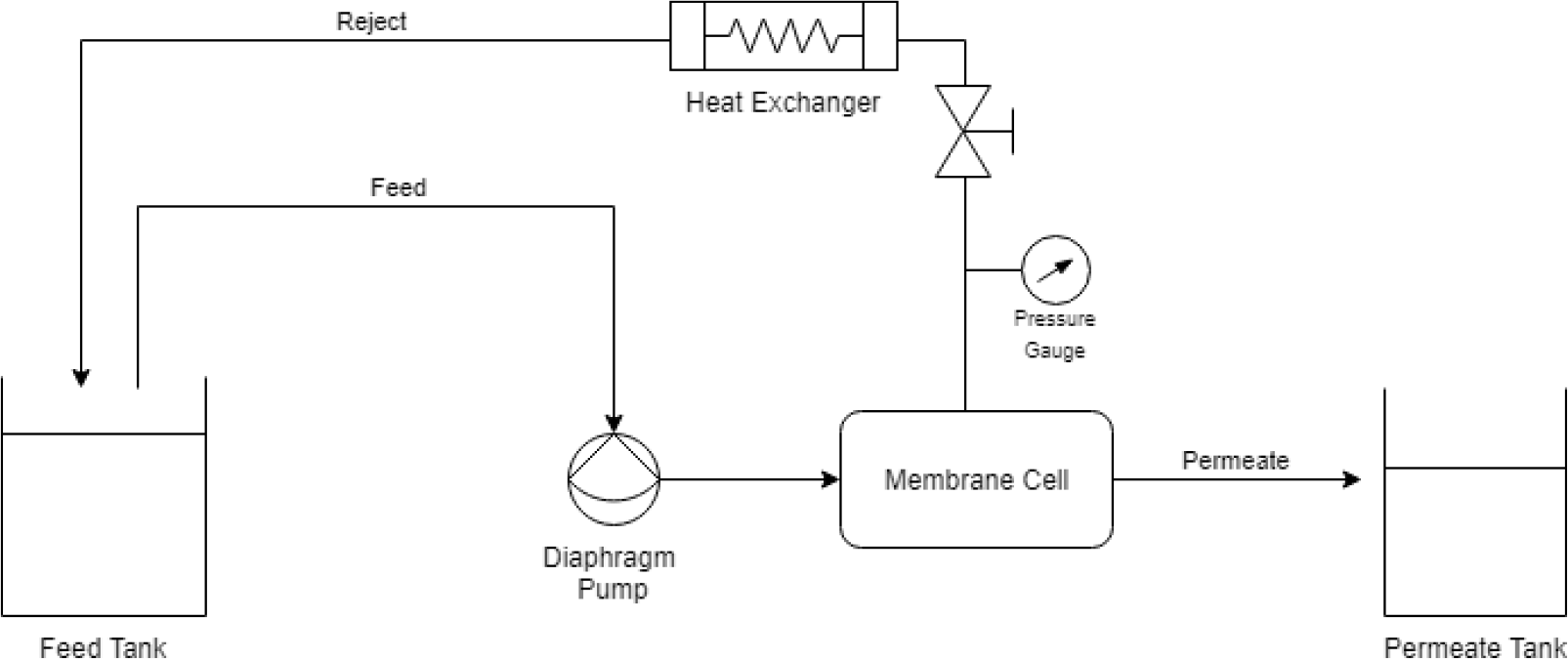
The experiments were carried out in a concentration mode of filtration, in other words the permeate stream was collected separately, while the reject was recycled back to the feed tank, thus reducing the volume of the feed and increasing the concentration. This filtration mode allows the study of membrane performance as contaminant and salts concentrations increase. In addition to emulating a method used to concentrate contaminant streams in order to more easily dispose of the reject using other techniques like advanced oxidation [23].
Before every experiment, the membrane was soaked for 24 hours in ultrapure MilliQ water to remove the preservative. The feed water (8L) which also consisted of MilliQ water, prior to spiking it with contaminants and salts, was used for a pre-filtration run to measure the pure water permeability of the membrane.
The PhACs were then added to the feed at a concentration of 10 mg/L, first individually each compound was added in addition to NaCl salt at three different concentrations. The salt was added to achieve a total dissolved solids (TDS) concentration of 300, 2000, and 8,000 ppm along with the control run that used distilled MilliQ water and had no salts added. After testing each pharmaceutical at 30 °C, which is the equilibrium temperature for the pump and the system, another run was completed at 20 °C, to study the effect of temperature.
Pressure was kept constant for the experiments at 130 psi as per manufacturer recommendations. The feed flow was also kept constant and measured using an F-550 panel mounted flow meter (Blue-White Industries Ltd., USA). The tangential flow velocity was chosen to be 0.5 m/s which is within the operating range of the pump and is comparable to the literature [13]. As for the permeate flux, it was measured by volumetrically collecting an amount of permeate using 25 ml and 10 ml graduated cylinders (ISOLAB Gmbh, Germany) with 95% accuracy, while a stopwatch recorded the time.
The flux was then calculated using the following equation [24]:
(1)
where J is the permeate flux (Lm-2h-1), A is the effective membrane area (m2), V is the volume of permeate collected (L) and t is the time recorded (hour). Experiments were continued until a volume reduction factor (VRF) of 2 is achieved. In other words, when the feed has been reduced to half of its volume, shown below:
where Vi and V care the initial feed volume and the final volume of the concentrate respectively. During each experiment, samples were collected from the feed and permeate streams and analyzed to measure pharmaceutical concentration, TDS, and pH. The concentrations of pharmaceuticals were measured in the feed and permeate, Cf and Cp respectively, and then the rejection percentage was calculated accordingly:
Finally, after each filtration run, the membrane unit was cleaned with 5% nitric acid (HNO3) and 1% sodium hydroxide (NaOH) solutions, and then MilliQ water to clear out any residues. The experimental conditions are summarized in the following Table 1.
Summarized experimental conditions
Variables | Operating Parameters | ||
---|---|---|---|
Membranes: | NF 270 | Pressure: | 130 psi |
Pharmaceuticals*: | CBZ – DCF – IBF | Cross Flow Velocity: | 0.5 m/s |
Salt Concentrations: | Distilled (<50 ppm) | Feed Volume: | 8 Liters |
300 ppm | Temperature: | 30 ± 0.5 °C | |
2,000 ppm | 20 ± 0.5 °C | ||
8,000 ppm |
* CBZ is carbamazepine, DCF is Diclofenac and IBF is ibuprofen
The membrane selected for this paper are the NF-270 membranes which are considered to be loose NF membranes, and have an ultrathin polyamide active layer on top of a porous polysulfone layer [13], [25]. The NF270 is manufactured by DOW Filmtec and was obtained via Sterlitech as flat sheet membrane coupons precisely cut to the respective cell size. The NF270 is a commonly used membrane in industry, usually to treat surface and ground waters. Its active layer is poly-piperazine amide, and it allows for high fluxes and has a medium salt and hardness passage [26]. As for the membrane properties, it is reported to have a negative charge, a pore size of 0.42 nm and a molecular weight cut off (MWCO) of 300 Daltons [27].
The selected pharmaceuticals, carbamazepine (CBZ; C15H12N2O), ibuprofen (IBF; C13H17NaO2), and diclofenac (DCF; C14H10C12NNaO2), are the compounds within the scope of this investigation. In fact they were selected because they are commonly used and are recognized as priority compounds especially in the developing world, in addition to having an estimated production volume of hundreds of tons annually and potential environmental and health risks [28], [29].
CBZ and the sodium forms of IBF and DCF were all obtained from Sigma-Aldrich (Germany). The compound properties are presented in Table 2, and they represent two pharmaceutical classes, different molecular weights, and charges in solution. The pharmaceuticals were dissolved in deionized water and sonicated to make stock solutions used to spike the feed with pharmaceuticals. The monovalent salt NaCl was obtained from Sigma-Aldrich with >98% purity and was used to mimic the effect of salts on the performance of the membranes. Methanol, acetonitrile, and formic acid were used as mobile phases for the analytical technique, in addition to the cleaning chemicals sulfuric acid and sodium hydroxide were all also obtained from Sigma-Adrich.
The properties of the representative pharmaceutical compounds [30]
PhAC | Carbamazepine (CBZ) | Ibuprofen Sodium (IBF) | Diclofenac Sodium (DCF) |
---|---|---|---|
Structure | |||
Pharmaceutical Class | Anticonvulsant | Analgesic (NSAID) | Analgesic (NSAID) |
Molecular Weight | 236.2 g/mol | 228.267 g/mol | 318.129 g/mol |
Molecular formula | C15H12N2O | C13H17NaO2 | C14H10C12NNaO2 |
Functional Groups | Carboxamide, Dibenzazepine derivative | Carboxylic Acid | Carboxylic Acid |
Log Kow* | 2.45 | 4.51 | 3.97 |
Charge in Solution | Neutral | Negative | Negative |
*Log Kow: Log n-octanol-water partition coefficient
Samples taken from the experiment were analyzed on the spot for conductivity and pH using a EUTECH CON11 conductivity meter from Thermo-Scientific, and a benchtop pH meter from Mettler-Toledo, respectively. Then the samples were placed in 2 mL vials for further pharmaceutical concentration analysis.
A High-Performance Liquid Chromatography (HPLC) system was used to determine the concentration of pharmaceuticals in solution. The HPLC system used the Agilent 1100Series LC system, equipped with a quaternary pump, an autosampler, and a diode array detector (DAD), and supported by an analytical workstation all supplied by Agilent Technologies (California, USA). The pharmaceuticals were separated using a reversed phase Supelco Discovery HS C-18 (5 mm, 25 cm × 4 mm ID) column along with a connected guard column (5 mm, 2 cm × 4 mm ID) both obtained from Sigma-Aldrich. Analytical calibration curves were constructed from prepared internal standards using the stock solutions with concentrations ranging from 1-15 mg/L. The methods used to test for the concentrations were adopted from previous studies and are shown in Table 3 [31].
HPLC Methods for the concerned pharmaceuticals
Name of Pharmaceutical | DCF | IBU | CBZ |
---|---|---|---|
Eluent | (Methanol) MeOH/0.1% formic acid in water (80:20, v/v) | (Acetonitrile) ACN/0.1% formic acid in water (65:35, v/v) | MeOH/0.1% formic acid water (70:30, v/v) |
Elution mode | isocratic mode | isocratic mode | isocratic mode |
Flow rate (mL/min) | 1 | 1 | 0.8 |
Injection volume (µL) | 25 | 50 | 30 |
Column temperature (°C) | 30 | 30 | 30 |
Detection wavelength (nm) | 275 | 196 | 235 |
Retention time (min) | 7.24 | 8.3 | 5.98 |
The results obtained from the nanofiltration experiments showed relatively high rejection values for ibuprofen (IBF) and carbamazepine (CBZ), while almost complete removal was shown for diclofenac (DCF). These results represent the experiments at 30 °C, because this was the equilibrium temperature for the system without cooling.
The rejection values for the pharmaceutical compounds in distilled water with the NF270 membrane are shown in Figure 2.
Rejection values for the three pharmaceutical compounds carbamazepine (CBZ), diclofenac (DCF) and ibuprofen (IBF) in distilled water with nanofiltration membrane NF270 at 30 °C taken at different times
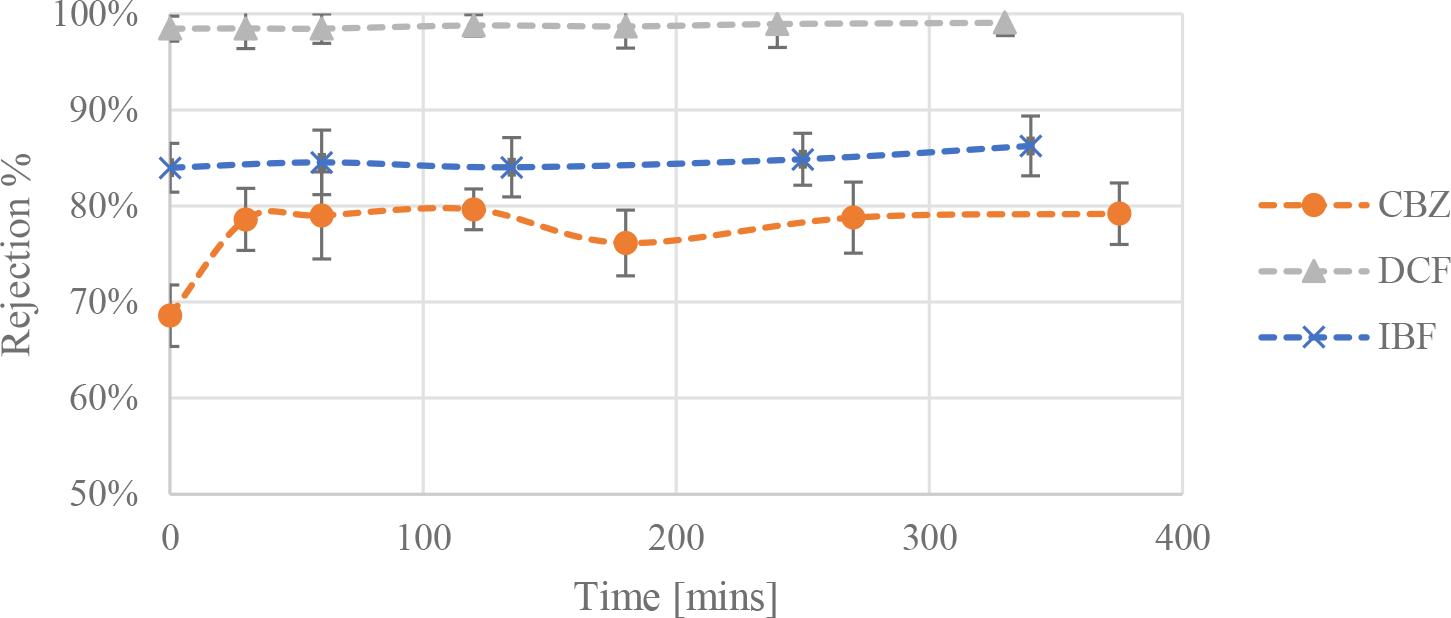
The steric hindrance effect, or size exclusion, is the dominant rejection mechanism for organic compounds like pharmaceuticals. The largest compound used was DCF with a molecular weight (MW) of 318 g/mol, well above the MWCO of NF270, and relatively large compounds like this one are expected to be rejected efficiently. Indeed, it can be seen that DCF had a consistently high rejection throughout the experiment. As for IBF, which is negatively charged with the lowest MW out of the three pharmaceuticals, the rejection increased slightly throughout the experimental runs. The role of electrostatic effects is more prominent since IBF has a MW less than or within close range of the membrane MWCO [32].
CBZ has an acid dissociation constant (pKa) value of 13.6, which implies that is it non-ionized and, at the experimental conditions investigated, is essentially a neutral organic solute when in solution, hence electrostatic effects are not expected to play a role when considering the rejection mechanisms [33]. Having a molecular weight close to the MWCO of the NF270 membrane, and a log Kow of 2.45, therefore steric hindrance and hydrophobic interactions will both influence the rejection of the compound. The initial increase in the rejection of CBZ could be attributed to the progressive adsorption of the compound on the surface of the membrane till it reached equilibrium and stabilized at around 77% rejection. It is likely that CBZ would adsorb on to the surface of the membrane and the pores using a hydrogen bond. The adsorption of CBZ on the NF270 membrane has been documented and it could lead to the overestimation of its rejection, due to the high concentration used in these experiments the membrane is expected to be saturated with CBZ within the first few minutes of the experiment [34]. In fact, mass balance calculations on the system showed that around 10% of the total mass of CBZ added was adsorbed on the membrane.
The average rejection values for the nanofiltration of the three PhACs using NF270 with distilled water at the 3 different salt concentrations are all presented in Figure 3. These values are averaged over time for the experiments, which were also performed at 30 °C.
Rejection values for the three pharmaceuticals compounds carbamazepine (CBZ), diclofenac (DCF) and ibuprofen (IBF), averaged over the duration of each experiment, in distilled water and at 3 different NaCl concentrations (300, 2,000, and 8,000 ppm) at 30 °C
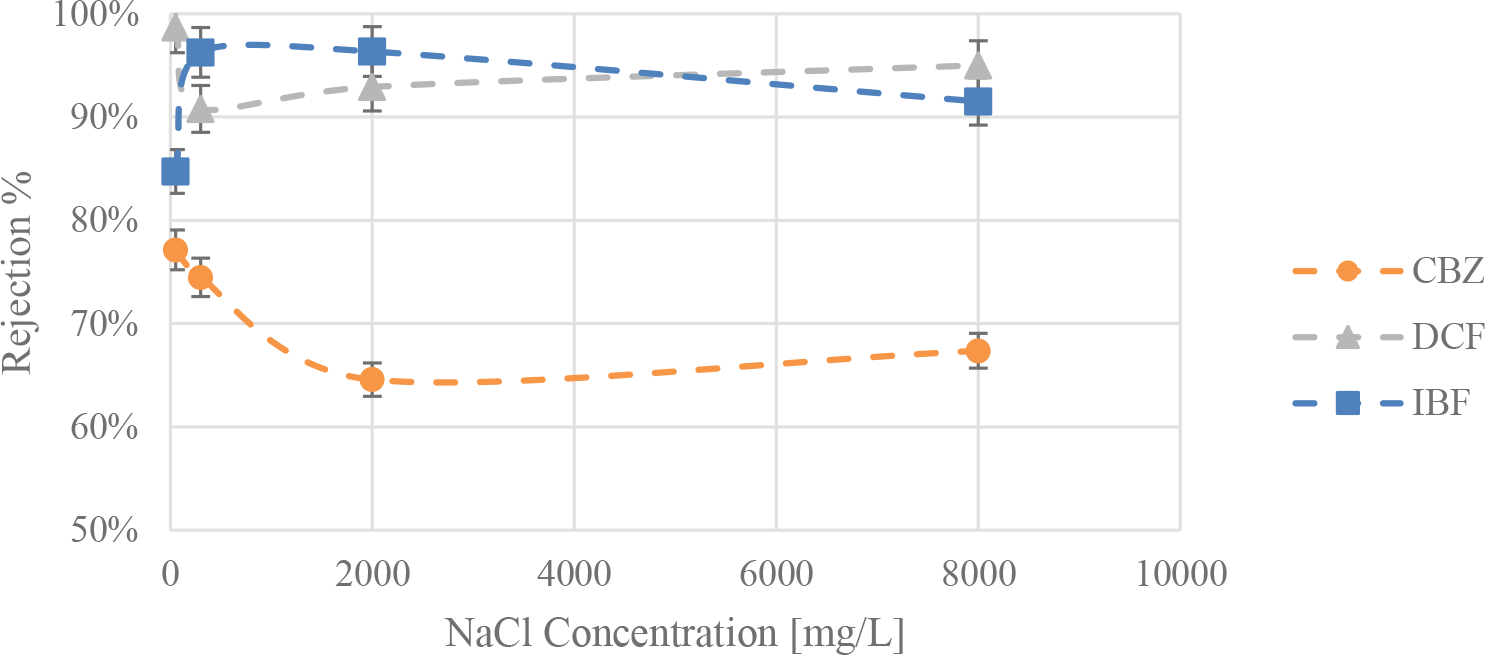
A decrease in the rejection of CBZ is observed with the increase of NaCl concentration, till the 8,000 ppm mark where a slight increase is shown. The results agree with the literature regarding the effects of salts in the nanofiltration of neutral organic solutes [35]. Two mechanisms might be simultaneously responsible for the drop in rejection rates, including the pore swelling effect as reported and the salting-out effect [36], [37]. CBZ has a low solubility in water, and adding salt to the mixture could very well cause its effective hydrated radius to decrease, making it more likely for the molecule to permeate the membrane. Both these mechanisms increase in significance with increasing salt concentrations; however, the threshold seems to point out that another effect occurs at high concentrations.
The higher volume of salt ions adsorbed on the membrane could slightly restrict the pores causing a flux decline and hindering the transport of CBZ through the membrane. This would explain the slight increase in rejection values at the 8000 ppm mark. It is interesting to note that this effect of NaCl on CBZ and the increase noted at the high concentration has been reported in literature and was attributed to the dehydration of the CBZ molecule allowing it to pass through the membrane more easily [38].
Alternatively, the high molecular weight of DCF and its negative charge had given the compound high rejection values when in distilled water. However, upon the addition of a low concentration of salt, a drop in rejection of 8% was observed, and the subsequent addition of more salts caused an increase in rejection. Figure 3 shows this effect at the three different experimental conditions. This case could be justified by the pore swelling phenomenon, as the addition of a low amount of salt (300 mg/L) could be enough for ion adsorption in the membrane pores and cause some repulsive forces inside the pores allowing them to swell. Upon increasing this concentration, it is speculated that a concentration gradient develops inside the pores causing the DCF molecules to also adsorb on the pores, however due to its size this could impact the pore size negatively causing a shrinkage or blockage of the pore, ultimately resulting in a better rejection.
Furthermore, Figure 4 shows the effect of increasing salt concentration on the instantaneous rejection of DCF during the experiment. The NF270 rejects NaCl at around 40%, and since the process is operated in concentration mode, this means that the salt concentration increases as the experiment progresses. A closer look shows that for the 300 ppm concentration experiment, the DCF rejection was at 88%; as the experiment approaches the end it is apparent that the salt concentration increases to 460 ppm, and DCF rejection value also increased to 92%. The same trend can be seen at higher concentrations, suggesting a possible positive relation between salt concentration and DCF rejection, when not considering the distilled water case. This shows that it is not only the average rejection value that is affected by the salts but also the instantaneous rejections during the experiment. This effect was not seen on the other pharmaceuticals.
Instantaneous rejection values for diclofenac (DCF) vs salt concentration in the 300 ppm experiment at 30 °C
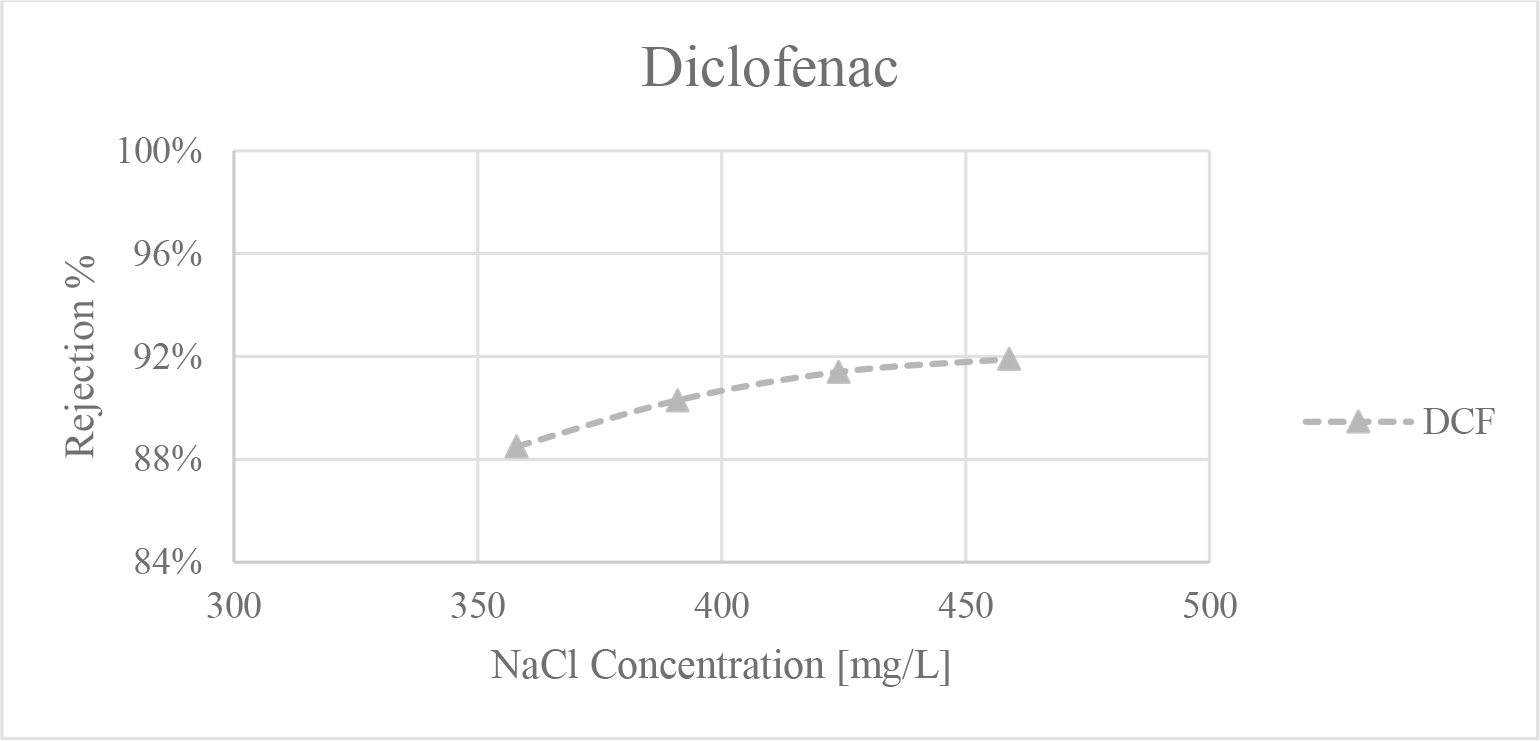
Finally, an increase in IBF rejection is observed when NaCl salt is added. Figure 3 shows an increase of 11% in the average rejection values as the salt concentrations are increased, until at the concentration of 8,000 ppm NaCl where the rejection drops, however still performing better than when no salts were added. Enhanced rejection for membrane processes when adding salt is not a usual phenomenon, since as discussed, salts more often cause reduction in rejection, flux, and overall performance of the membrane.
An increase in ionic strength of the feed solution decreases the effective charge density of the membrane, and this allows the passage of more cations to the permeate side. This creates a charge imbalance and demands anions to permeate through the membrane to maintain electroneutrality of the solution thus causing a competition between the anions. This result was present in a study where the effect of ammonium salts on the nanofiltration of the amino acid glutamate was discussed, and an increase in the rejection of the solute was reported. This increase is attributed to co-ions competition, and in this case, it represents the competitive transmission of chloride ions to the permeate since Cl- has higher mobility and less charge than the glutamate amino acid, hence increasing the rejection of the solute [39].
In fact IBF rejection in nanofiltration was reported to increase with increasing NaCl concentration [22]. The drop in rejection was also observed at around 5,000 ppm; this means there is a threshold where either the positive effect of the salt on rejection starts to become less significant or another negative effect starts to counteract the former. The mechanism reported that was responsible for this increase in rejection was anion competition. Chloride ions have a higher diffusivity than IBF molecules, thus allowing their transport through the membrane more easily. The results presented in Figure 3 can be explained by this co-ion competitive mechanism as well.
However, in another study an enhanced rejection of pesticides was reported in tap water vs distilled water. The reasoning behind the results was the interactions of ions and the membrane. The ions in tap water adsorb on the membrane surface or inside the pores, thus the pores become narrower and pesticides are rejected more efficiently [40]. Even though this is not in accordance with the pore swelling theory, which speculates that adsorbed ions cause repulsive forces that increase the membrane pore size, however it is possible that these ions along with the large pesticide molecules could cause pore narrowing.
The threshold observed could be the result of either pore swelling starting to take effect due to the high volume of ions adsorbed on the membrane or a balanced electroneutrality. IBF would no longer have a disadvantage to permeate the membrane at this balanced point, however the rejection still seems to be better at this point rather than with the distilled water. This threshold can also be due to the dehydration effect and further experiments at higher concentration could help make this clearer.
Similar to the experiments changing the salinity, which were conducted at 30 °C, two additional trials were conducted at 20 °C. The average rejections (%) obtained in both attempts were plotted along with the previous attempts (at 30 °C) with respect to NaCl concentration (ppm). The results are shown below in Figure 5.
The effect of temperature on the average rejection values for the three pharmaceuticals, in distilled water and at 3 different NaCl concentrations (300, 2,000 and 8,000 ppm): (a) Carbamazepine; (b) Ibuprofen; (c) Diclofenac
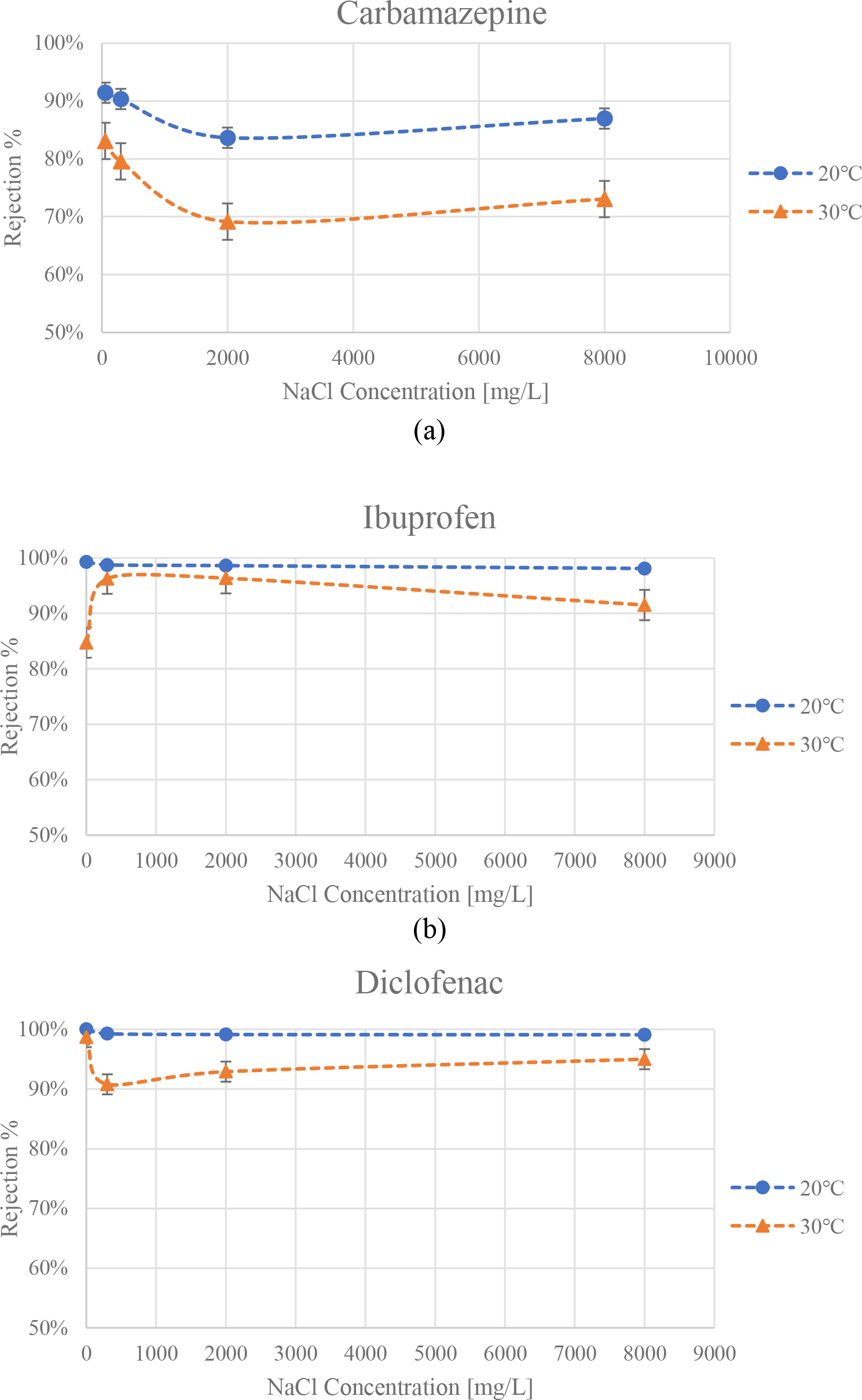
The same trend observed for the change in average rejection of CBZ versus varying NaCl concentrations at 30 °C was also obtained at 20 °C as seen in Figure 5. The average rejection hit its peak of 91.4% in the distilled water solvent experiments, and its minimum at 2000 ppm NaCl with an average rejection of 83.6%. There exists the same general increasing trend for NaCl concentrations higher than 2000 ppm. The major difference between the two controlled temperatures was an overall higher rejection of carbamazepine in all experiments conducted at 20 °C compared to those done at 30 °C. The average increase in rejection ranged from 8% up to 14%.
This increase in rejection could be explained using two linked concepts. The expected dominant rejection mechanism for CBZ was determined to be size exclusion, as referred to in the previous section, given that carbamazepine is an uncharged molecule and is rarely affected by the electrostatic forces on the surface of the membrane. In addition, at higher temperatures, the active layer of the membrane undergoes thermal expansion which increases the pore size of NF membranes and thus decreases their rejection [41]. Therefore, the prominently dominant mechanism, size exclusion, is directly affected by the change in temperature. At lower temperatures, the smaller pore size limits the passage of CBZ particles through the membrane leading to higher rejection rates. This phenomenon may also be observed in the IBF and DCF experiments.
The rejection of IBF decreases as the salt concentration increases for both control temperatures; however, there is an apparent difference in behaviour between the two sets. The experiments conducted at 30 °C were affected by the change in salt concentration more than those of 20 °C, as shown in Figure 5. As previously discussed, this is mainly due to the effect that the ionic strength of the feed has on the electrostatic interactions between the charged IBF molecules and the membrane. The effects of this mechanism are more noticeable at higher temperatures due to the expansion of the pores which seems to greatly affect the relatively small IBF particles. At lower temperatures, it was concluded that size exclusion was the dominant mechanism; on the other hand, as the pores expanded with the increase in temperature, the electrostatic interactions started to become the more dominant mechanism.
In addition to the behavioural differences, another difference between IBF and CBZ includes the magnitude in which the change in temperature affects the rejection. The average difference between the rejections for CBZ at 30 °C and 20 °C is about 12% while the average difference between the IBF temperatures is about 6%. This coincides with Dang et al. study [42] which stated that neutral PhACs are dominantly affected by the increase in pore size. It concludes that this is due to the presence of the electrostatic repulsion mechanism which is minimal for neutral PhACs. These conclusions were consistent with the results obtained from these experiments.
The rejection of DCF at 20 °C was very high according to detection limits, leaving traces in the resulting permeate and getting a rejection value above 99%. While DCF rejection was high regardless of the temperature, we can see that the lower temperatures increased the rejection further solidifying the claim that lower temperatures increase rejection values. Moreover, the effect of salt mechanisms was not noticeable in the experiments at 20 °C. The pore size could be the limiting factor here again since DCF’s molecular weight is greater than the membrane MWCO, and size exclusion was possibly the dominant mechanism once again at the lower temperature, similar to the case with IBF.
Many factors that play a role in the nanofiltration of contaminants and PhACs make it complicated to fully understand the process with its nuances. Although the bulk of the discussion of this work is speculative in nature, these speculations pave the way for further studies with other control variables which may lend further support to these explanations or refute these speculations but nevertheless, advance our understanding of mechanisms involved in the removal of PHACs by nanofiltration. Some factors to be investigated along with salt concentration include solution pH and the presence of divalent ions, for a more complete understanding of solution chemistry and its role in the process. Furthermore, experiments on different membranes and a mixture of pharmaceuticals are necessary to supplement the evidence for the removal mechanisms involved.
In this work, a bench-scale membrane system was used to evaluate the removal of three pharmaceuticals by NF membranes at different salt concentrations and temperatures. The rejection values in distilled water for the nanofiltration of CBZ, DCF, and IBF were 77%, 98% and 85% respectively. It was determined that a combination of mechanisms were responsible for the rejection of these pharmaceuticals. Size exclusion was the dominant mechanism in all cases, whereas electrostatic repulsions enhanced the rejection of the charged molecules, IBF and DCF, and adsorption increased CBZ rejection.
The effect of the monovalent salt NaCl on the filtration of the pharmaceuticals was found to be dependent on the compound properties. For nanofiltration, the increase in salt concentration enhanced the retention of the smallest charged compound IBF and decreased the rejection values for CBZ and DCF. Different mechanisms were responsible for the changes in rejection, including the pore swelling, salting-out, and competitive ion transmission effects. The experimental results also revealed a threshold at 8000 ppm where properties start to change for IBF and CBZ.
Performing the experiments at a lower temperature of 20 °C caused the rejection values to increase in all pharmaceuticals and at all salt concentrations. The decrease in temperature is likely to shrink the pore size of the membrane leading to better hindrance of the molecules regardless of the salt effects. The polymer expansion of the membrane at high temperatures changes pore size values.
- , World Health Organization, Pharmaceuticals in drinking-water, 2012
Occurrence of drugs in German sewage treatment plants and rivers ,Water research , Vol. 32 (11),pp 3245-3260 , 1998, https://doi.org/https://doi.org/10.1016/S0043-1354(98)00099-2
, Riverbank Filtration: A sustainable process to attenuate contaminants during drinking water production ,Journal of Sustainable Development of Energy, Water and Environment Systems , Vol. 6 (1),pp 150-161 , 2018, https://doi.org/http://dx.doi.org/10.13044/j.sdewes.2013.01.0009
, Widespread sexual disruption in wild fish ,Environmental science & technology , Vol. 32 (17),pp 2498-2506 , 1998, https://doi.org/https://doi.org/10.1021/es9710870
, Diclofenac residues as the cause of vulture population decline in Pakistan ,Nature , Vol. 427 ,pp 630-633 , 2004, https://doi.org/https://doi:10.1038/nature02317
, Pharmaceuticals as emerging contaminants and their removal from water ,Chemosphere , Vol. 93 (7),pp 1268-1287 , 2013, https://doi.org/https://doi.org/10.1016/j.chemosphere.2013.07.059
, - , Desk based review of current knowledge on pharmaceuticals in drinking water and estimation of potential levels, 2007
Elimination of pharmaceuticals in sewage treatment plants in Finland ,Water Research , Vol. 41 (5),pp 1001-1012 , 2007, https://doi.org/https://dx.doi.org/10.1016/j.watres.2006.12.017
, Potential of concentrating solar power plants for the combined production of water and electricity in MENA countries ,Journal of Sustainable Development of Energy, Water and Environment Systems , Vol. 1 (2),pp 122-140 , 2013, https://doi.org/http://dx.doi.org/10.13044/j.sdewes.2013.01.0009
, Assessing potential of nanofiltration and reverse osmosis for removal of toxic pharmaceuticals from water ,Journal of Water Process Engineering , Vol. 25 ,pp 195-204 , 2018, https://doi.org/https://doi.org/10.1016/j.jwpe.2018.08.002
, A critical review on membrane separation processes applied to remove pharmaceutically active compounds from water and wastewater ,Journal of water process engineering , Vol. 26 ,pp 156-175 , 2018, https://doi.org/https://doi.org/10.1016/j.jwpe.2018.10.010
, - , Nanofiltration, Advanced Membrane Technology and Applications (Li, N.N., Fane, A.G., Winston Ho, W.S. and Matsuura T., ed.), 2008
Nanofiltration for wastewater reuse: Counteractive effects of fouling and matrice on the rejection of pharmaceutical active compounds ,Separation and Purification Technology , Vol. 133 ,pp 313-327 , 2014, https://doi.org/https://doi.org/10.1016/j.seppur.2014.07.007
, Rejection of pharmaceuticals in nanofiltration and reverse osmosis membrane drinking water treatment ,Water Research , Vol. 42 (12),pp 3601-3610 , 2008, https://doi.org/https://doi.org/10.1016/j.watres.2008.05.020
, Nanofiltration and ultrafiltration of endocrine disrupting compounds, pharmaceuticals and personal care products ,Journal of Membrane Science , Vol. 270 (1-2),pp 88-100 , 2006, https://doi.org/https://doi.org/10.1016/j.memsci.2005.06.045
, Impact of natural water colloids and cations on the rejection of pharmaceutically active and endocrine disrupting compounds by nanofiltration ,Journal of membrane science , Vol. 450 ,pp 272-281 , 2014, https://doi.org/https://doi.org/10.1016/j.memsci.2013.09.017
, Pollution from drug manufacturing: review and perspectives ,Philosophical Transactions of the Royal Society B: Biological Sciences , Vol. 369 (1656),pp 20130571 , 2014, https://doi.org/https://doi.org/10.1098/rstb.2013.0571
, Environmental loadings of Active Pharmaceutical Ingredients from manufacturing facilities in Canada ,Science of The Total Environment , Vol. 646 ,pp 257-264 , 2019, https://doi.org/https://doi.org/10.1016/j.scitotenv.2018.07.240
, Nanofiltration for the removal of norfloxacin from pharmaceutical effluent ,Journal of environmental chemical engineering , Vol. 6 (5),pp 6147-6153 , 2018, https://doi.org/https://doi.org/10.1016/j.jece.2018.09.034
, Removal of emerging contaminants from wastewater using nanofiltration for its subsequent reuse: Full–scale pilot plant ,Journal of Cleaner Production , Vol. 214 ,pp 514-523 , 2019, https://doi.org/https://doi.org/10.1016/j.jclepro.2018.12.297
, An innovative of aerobic bio-entrapped salt marsh sediment membrane reactor for the treatment of high-saline pharmaceutical wastewater ,Chemical Engineering Journal , Vol. 295 ,pp 317-325 , 2016, https://doi.org/https://doi.org/10.1016/j.cej.2016.03.046
, Influence of inorganic salt on retention of ibuprofen by nanofiltration ,Separation and Purification Technology , Vol. 189 ,pp 382-288 , 2017, https://doi.org/https://doi.org/10.1016/j.seppur.2017.08.035
, Coupling of membrane filtration and advanced oxidation processes for removal of pharmaceutical residues: a critical review ,Separation and Purification Technology , Vol. 156 ,pp 891-914 , 2015, https://doi.org/https://doi.org/10.1016/j.seppur.2015.09.059
, CFD modeling of permeate flux in cross-flow microfiltration membrane ,Journal of Membrane Science , Vol. 255 (1),pp 23-31 , 2005, https://doi.org/https://doi.org/10.1016/j.memsci.2005.01.024
, Swelling and morphology of the skin layer of polyamide composite membranes: an atomic force microscopy study ,Environmental science & technology , Vol. 38 (11),pp 3168-3175 , 2004, https://doi.org/https://doi.org/10.1021/es034815u
, - https://www.dow.com/en-us/markets-and-solutions/products/DOWFILMTECNanofiltration4Elements/DOWFILMTECNF2704040, Available from[Accessed: 22-July-2018] ,
Elucidating the rejection mechanisms of PPCPs by nanofiltration and reverse osmosis membranes ,Industrial & Engineering Chemistry Research , Vol. 53 (16),pp 6798-6806 , 2014, https://doi.org/https://doi.org/10.1021/ie500114r
, Environmental risk analysis and prioritization of pharmaceuticals in a developing world context ,Science of The Total Environment , Vol. 557-558 ,pp 31-43 , 2016, https://doi.org/https://doi.org/10.1016/j.scitotenv.2016.03.023
, Application of nanofiltration for the removal of carbamazepine, diclofenac and ibuprofen from drinking water sources ,Journal of environmental management , Vol. 127 ,pp 177-187 , 2013, https://doi.org/https://doi.org/10.1016/j.jenvman.2013.04.036
, - National Center for Biotechnology Information. PubChem Compound Database; CID=2554, https://pubchem.ncbi.nlm.nih.gov/compound/2554, [Accessed: 22-July-2018]
The fate of selected pharmaceuticals in solar stills: Transfer, thermal degradation or photolysis? ,Science of The Total Environment , Vol. 574 ,pp 583-593 , 2017, https://doi.org/https://doi.org/10.1016/j.scitotenv.2016.09.082
, Removal of organic micro-pollutants during drinking water treatment by nanofiltration and reverse osmosis ,Desalination and Water Treatment , Vol. 13 (1-3),pp 226-237 , 2010, https://doi.org/https://doi.org/10.5004/dwt.2010.1063
, Effects of organic, biological and colloidal fouling on the removal of pharmaceuticals and personal care products by nanofiltration and reverse osmosis membranes ,Journal of Membrane Science , Vol. 542 ,pp 342-351 , 2017, https://doi.org/https://doi.org/10.1016/j.memsci.2017.08.023
, Pharmaceutical Retention Mechanisms by Nanofiltration Membranes ,Environmental Science & Technology , Vol. 39 (19),pp 7698-7705 , 2005, https://doi.org/https://doi.org/10.1021/es0507665
, Effects of pH and salt on nanofiltration—a critical review ,Journal of Membrane Science , Vol. 438 ,pp 18-28 , 2013, https://doi.org/https://doi.org/10.1016/j.memsci.2013.03.029
, Nanofiltration of multi-component feeds. Interactions between neutral and charged components and their effect on retention ,Journal of Membrane Science , Vol. 247 (1-2),pp 11-20 , 2005, https://doi.org/https://doi.org/10.1016/j.memsci.2004.05.022
, Influence of salts on the rejection of polyethyleneglycol by an NF organic membrane: Pore swelling and salting-out effects ,Journal of Membrane Science , Vol. 347 (1),pp 174-182 , 2010, https://doi.org/https://doi.org/10.1016/j.memsci.2009.10.021
, Influence of seasonal and operating conditions on the rejection of pharmaceutical active compounds by RO and NF membranes ,Desalination , Vol. 277 (1),pp 250-256 , 2011, https://doi.org/https://doi:10.1016/j.desal.2011.04.029
, Separation of l-glutamine from fermentation broth by nanofiltration ,Journal of Membrane Science , Vol. 222 (1),pp 191-201 , 2003, https://doi.org/https://doi.org/10.1016/S0376-7388(03)00290-4
, Removal of pesticides by nanofiltration: effect of the water matrix ,Separation and Purification Technology , Vol. 382 (2),pp 163-172 , 2004, https://doi.org/https://doi.org/10.1016/j.seppur.2003.11.003
, Temperature effects on sieving characteristics of thin-film composite nanofiltration membranes: pore size distributions and transport parameters ,Journal of membrane science , Vol. 223 (1-2),pp 69-87 , 2003, https://doi.org/https://doi.org/10.1016/S0376-7388(03)00310-7
, The effects of feed solution temperature on pore size and trace organic contaminant rejection by the nanofiltration membrane NF270 ,Separation and Purification Technology , Vol. 125 ,pp 43-51 , 2014, https://doi.org/https://doi.org/10.1016/j.seppur.2013.12.043
,