Many industrial processes generate waste gases. The composition of waste gas (WG) is dependent on many aspects (such as the type of product produced in a process plant, used technology, etc.). However, it usually contains harmful substances, therefore an appropriate cleaning technology must be employed to prevent the emissions to the environment. For example, the WGs produced in chemical and petrochemical plants often contain Volatile Organic Compounds (VOC) or carbon monoxide (CO), which are harmful to human health and the environment. The various production processes, such as paint production, oil refinery, organic acid production and others, generate air polluted with some amount of VOC and/or CO. Further, a huge amount of the air contaminated with VOC is generated in printing shops, especially in the automotive industry. This polluted air (as an industrial WG) must be treated before its discharge to the environment.
To control the VOC and CO emissions contained in WGs at small concentration, the thermal oxidation technology has been found an effective and reliable abatement technique which commonly reaches the pollutant removal efficiency of over 99%. It is applied to processing large quantities of WG with a low concentration of combustible substances (VOC/CO). The pollutant thermal oxidation (decomposition) is in principle a contaminant flameless ignition which results in a sudden temperature increase of processed waste gas and pollutant thermal decomposition to carbon dioxide (CO2) and water. Thermal oxidation takes place in a combustion chamber (CC) at high temperatures commonly ranging between 730–850°C with necessary residence time while flue gas (FG) is produced [1]. Maintaining the prescribed temperatures in the CC for the pollutant ignition requires intensive energy demand provided by a supplemental fuel, which is associated with high operating costs. In order to improve the economic aspect of the unit´s operation, the waste heat contained in a generated flue gas is typically used for energy purposes (e.g., steam generation) and technology purposes (WG preheating before its entering CC to reduce the supplemental fuel demand) through series of individual waste heat recovery exchangers, i.e., through the Heat Recovery System (HRS). Further, FG cleaning technology (filters, absorbers, scrubbers…) could be employed to remove acid compounds, solid particles, or other gaseous pollutants if necessary. A thorough review of the air pollution control techniques including the abatement of VOC pollution was published by Schnelle et al.[1]. A simplified technological layout of such waste gas to energy (WGtE) unit is illustrated in [Figure 1].
Standard waste gas-to-energy unit
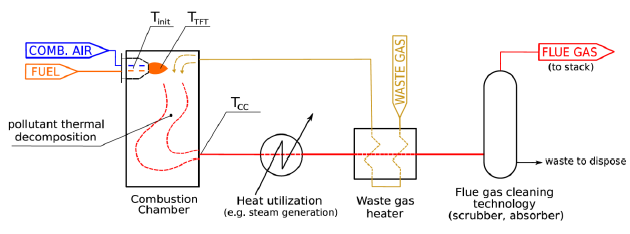
Due to the continuous fuel price rise, there is an effort to improve the existing WGtE units in terms of the units´ fuel demand reduction, i.e., performing the Energy Retrofit (ER). As suggested above, fuel saving in a standard WGtE unit is achieved by preheating the WG stream before its thermal processing in the CC. As the WG preheating is realized by utilization of the waste heat contained in the flue gas (i.e., through some heat exchangers from HRS), the fuel savings could be reached by the Integration and Intensification of the existing HRS (Klemeš et al. [2]).
A great effort has been put in the last decades into the research of increasing the process efficiency in order to reduce the external utility demand (fuel, water resources) with respect to minimum investment costs. Most current techniques and methodologies focused on effective retrofit and intensification of heat exchanger network (HEN) are inspired by or a direct outcome of the initial works and principles of Process Integration dated in the late 1970s with the discovery of the Pinch Analysis concept (Linnhoff and Flower [3]). Akpomiemie and Smith [4], for example, brought a novel methodology for the cost-effective heat transfer enhancement in the existing HEN. Further, Jiang et al. [5] discussed a possibility of the HEN retrofit by reusing the existing heat exchangers. To improve the quality of HEN retrofit design, Lai et al. [6] also considered the influence of physical distance between heat exchangers, pressure drop and available space for equipment. The advantages of an industrial plant´s retrofit on a practical example of an oil refinery were published by Marton et al. [7]. The comprehensive summary of traditional and modern methods for HEN retrofit was published by Klemeš et al. [8].
The Process Integration is not limited only to the topic of heat exchange in HENs. Thermal energy contained in the process streams is only a part of the total energy content. Pressure levels of the process streams, for example, reflect the necessity of mechanical work input, i.e., the pumping power, which results in considerable electricity demand. Industrial processes can be designed or retrofitted with respect to minimization of both, the heat duty and power duty. Fu et al. [9] presented the advantages of simultaneous work and heat integration applying graphical and mathematical approaches. Deng et al. [10] proposed a method for utilization of residual pressure energy based on Pinch Analysis. In summary, Yu et al. [11] published a review of work and heat exchange networks (WHENs) reflecting the current state-of-the-art.
There are available many analytical methods enabling energy recovery improvement within the studied process, but the process integration of large industrial processes represents a complex problem, where mathematical programming is applied. Wissocq et al. [12], for example, proposed a method based on a mixed-integer-linear-programming (MILP) model for an optimal design of large industrial plants enabling a suitable technology selection. Linear models´ applications require many simplifications, which is associated with a reduction in the results´ accuracy. To overcome this shortcoming, Nemet et al. [13] proposed a two-stage method incorporating the MILP model in combination with a mixed-integer-nonlinear-programming (MINLP) model. Additionally, Santos et al. [14] applied a MINLP model to perform the process optimization in terms of heat and work duty minimization.
Even though the presented advanced methods can be used to reduce the energy demand of industrial processes, their achievable energy efficiency is still thermodynamically limited. Therefore a reliable and sustainable heat and power source is necessary. Nowadays a number of modern technologies are available for the effective production of heat and power. For example, there is an effort to retrofit the traditional energy producers, such as coal-fired plants, by cost effective co-generation technology introduction. The main goal is a deeper implementation of renewable energy sources. The potential of introducing co-generation blocks producing power from biomass to the existing coal-fired plant was studied by Kalina [15]. Thermal and economic optimization of this technological solution was performed by Tańczuk et al. [16]. Furthermore, the energy sources could be used to generate power and to provide heating and cooling simultaneously. Katsaros et al. [17], for example, proposed such a tri-generation system based on municipal waste gasification.
As mentioned above, there is a continuous effort to implement more renewable energy sources (e.g., solar and wind energy) to the current energy system. However, energy production from these sources is unstable, which can cause stability issues in the power grid. The power grid capacity for acceptance of various renewable energy sources was studied by Taseska-Gjorgievska et al. [18]. Further, Morel et al. [19] proposed a potential of power grid capacity increase by implementation of batteries and exploiting the kinetic energy of wind turbines.
Various types of industrial and municipal wastes represent another significant energy sources with great potential to cover a part of heat and power consumption. Compared to discussed solar and wind energy, an advantage of industrial plants processing waste is their relatively stable energy generation. On the other hand, the main disadvantage is the necessity of supplemental fuel for thermal waste processing. However, in case of WGtE units, the amount of supplemental fuel can be significantly reduced by improving the waste heat recovery (as discussed above). The ER of WGtE units can therefore reduce operational costs due to the fuel demand reduction, while the energy production (e.g., steam production, thermal oil heating, etc.) is maintained.
However, from the above description of WGtE units, it is obvious that the ER of the WGtE process cannot employ these sophisticated HEN retrofit strategies since HRS of WGtE process has substantially different specificity than the standard process HEN, especially that HRS does not contain any Utility Path. Even though some methods are available for an efficient retrofit of HENs not containing Utility Path (such as Jegla and Freisleben [20]), none of these methods can be applied to the case of WGtE units, as their HRS does not allow creating a new Utility Path due to the absence of hot and cold utility. So, for ER of WGtE units, it is necessary to apply some specific retrofit approaches focusing on the flue gas stream as a waste heat source and taking into account the specificity of the WGtE process. As no suitable approaches are currently available for the ER of the WGtE units, this research work presents a decision making and evaluating method, which is called the Shifting Flue Gas Line (SFGL) method, that represents a conceptual design method for ER of WGtE units. The SFGL method will be presented in detail in this article. It is a method developed for the retrofit of WGtE units in order to reduce their energy demand, while high pollutant removal efficiency is maintained.
An accurate evaluation of fuel savings is another important aspect of the proper ER of WGtE units. It could be performed by the process non-linear simulation using commercial software. This advanced system modelling might however be a drawback in terms of acquisition costs and also in terms of applicability of WGtE process retrofit targeting, where conceptual modifications enabling the desired energy savings should be proposed. Therefore, the non-linear simulation does not have to be necessarily convenient during the targeting and conceptual design stage.
Freisleben and Jegla [21] presented a simple and fairly accurate method to calculate the fuel savings of units for waste thermal processing which does not require an advanced non-linear system modelling. This analytical method is based only on the initial temperature of the supplemental fuel/oxidizer (Tinit), the Theoretical Flame Temperature (TTFT), the fuel Lower Heating Value (LHV), and the flue gas temperature (TCC) prescribed for sufficient pollutant removal (see [Figure 1]). This calculation procedure was applied in the developed SFGL method presented in this paper.
It should be emphasized that the purpose of the developed method is to enable fairly accurate WGtE units modelling and a specific ER evaluation and not that of finding an optimal solution of ER. It is an analytical approach, which does not require introduction of advanced mathematical models and optimization approach presented above.
The developed SFGL method is described in the paper and further practically introduced by its application to a case study of a specific WGtE unit´s Energy Retrofit. The obtained results are then compared to the non-linear simulation to verify the accuracy of the developed method. The non-linear simulation was carried out in software CHEMCAD (in the latest version CHEMCAD 7) from Chemstations Inc. [22] in combination with software Xchanger Suite® from Heat Transfer Research Inc. (HTRI) [23].
As mentioned in the Introduction section, the Shifting Flue Gas Line (SFGL) method is designed for ER of standard WGtE units, i.e., the units for thermal processing of waste gases, specifically waste gases containing combustible substances such as VOC or CO. It benefits from a relatively simple WGtE unit technological arrangement, where only a small number of WG processing and heat recovery equipment is employed. The method enables estimating several key features, such as the following:
Flue gas heat recovery efficiency and Energy Retrofit targeting . The amount of the flue gas waste heat which is currently lost is calculated and could be utilized to reduce the supplemental fuel demand.The conceptual design of the technological modifications that are required to achieve the desired energy savings.Process parameters re-evaluation , such as temperature profiles and flowrates of process streams and basic parameters of newly added or modified equipment (as e.g., heat exchangers´ heat loads).
The main advantage of the developed method is its practicability and simplicity. It is based on a linear model of studied processes that employs a limited number of WG processing and FG heat recovery equipment, so the method can be performed by simple desk calculation.
The main drawback of the developed method is its limited applicability. It cannot be effectively applied to the wide range of industrial processes as it was tailor made for WGtE units using their specific characteristics, which are discussed further in the paper.The SFGL method is in principle a simple calculation procedure supported by graphical representation of performed modifications to promote the designer’s interactivity when performing the ER.
The key equipment is an industrial furnace where the pollutant thermal oxidation takes place. The SFGL method is therefore inspired by analytical approaches for Furnace Heat Integration, especially by flue gas line representation (or flue gas temperature-enthalpy profile). The FG line was first proposed by Linnhoff and de Leur [24] and later justified by Stehlík et al. [25]. Following these works, Jegla et al. [26] then introduced the specific manipulation with the FG profile for efficient furnace retrofit. Inspired by this FG line operation, the SGFL method presented here is divided into several systematic stages, which are described below.
The Energy Retrofit procedure starts with a preparation stage, where all key parameters of the existing unit are obtained. It consists of several points:
Process streams and equipment characteristics including the temperatures, pressures, composition, average specific heat capacities and flowrates of all streams present in the current WGtE unit (FG, WG, steam, hot water or another energy medium…). Further, the characteristics of applied heat exchangers are obtained, like type, geometry, and heat loads.Supplemental fuel/oxidizer characteristics , which includes a fuel Lower Heating Value (LHV), Theoretical Flame Temperature (TTFT), initial temperature (Tinit), and mixing ratio (K) of oxidizer/fuel mixture being combusted in WGtE unit´s furnace.Drawing of the temperature-enthalpy diagram containing temperature-enthalpy profiles (–hereafter referred to as just profiles) of all (hot and cold) present streams. An example of such a diagram, corresponding to the unit technological layout presented in [Figure 1], is shown in [Figure 2].
WGtE units contain typically only one hot stream, which is the FG coming out of CC. In practice, the splitting of the FG stream is not applied in WGtE units due to the additional investment costs of the FG duct and decreased operational reliability. The FG profile (or FG line) is plotted in an interval between a CC outlet temperature (TCC) and a stack temperature (Tstack). This interval represents the amount of heat, which is utilized in the existing unit.The FG line is then linearly extrapolated to the dew point temperature (TDP), where the condensation is expected to occur.
The interval between Tstack and TDP represents an approximate value of the amount of heat with a potential to be utilized but is currently lost (Qloss). TDP is chosen as a limit temperature to avoid the generation of the condensed substances (especially acidic) in FG to prevent the equipment from corrosion and damage.
The cold stream profiles are not combined into a Cold Composite Curve (unlike the standard practice in the traditional Process Integration approach based on Pinch Analysis [2]), but they are plotted separately. As the FG stream is not usually split, this graphical representation (shown in [Figure 2]) reflects the actual heat exchanger arrangement in the existing HRS, where the FG heat is used at first for a steam generation (Qsteam) and then for WG preheating (QWG).
Temperature-enthalpy diagram of existing Waste GastoEnergy unit
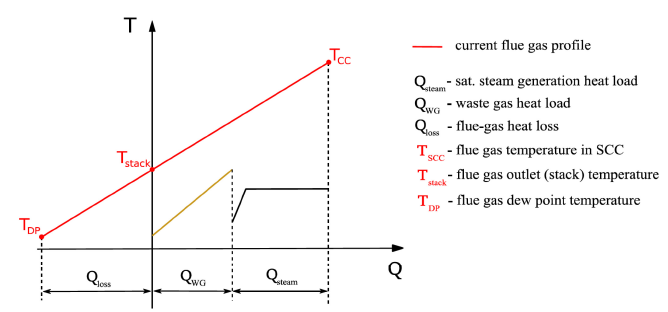
As mentioned in the Introduction, the heat contained in FG is commonly used to preheat the WG stream in order to reduce the unit´s energy demand. For that reason, the WG heater is commonly employed as shown in [Figure 1]. The WG preheating enhancement is recommended as the first thing to consider while the ER of the existing WGtE unit is desired. This could be performed, for example, by increasing heat transfer area (usually relatively costly), or implementation of heat transfer intensification technology to the existing WG heater, which provides a cheap solution to reach the exchanger enhancement. The selection of appropriate intensification technology depends on several aspects, such as a type and geometry of the existing WG heater and media process parameters, such as temperatures, fouling sensitivity, or allowed pressure drop. The analysis and comparison of the most suitable and efficient heat transfer enhancement technologies for tube WG heater was performed, for example, in [27].
After a suitable method for WG preheating intensification is selected, the fuel saving could be evaluated according to the following steps:
Heat transfer increase evaluation . Based on the selected enhancement technology or method, the intensified heat transfer (Qint) to the WG stream is evaluated.Fuel saving calculation . It is performed using the equations (1) and (2) below [21].
(1)
(2)
FHVCC defines the fuel energy content utilizable to keep the high temperature inside CC, Δfs is an achieved fuel saving and nc is a correction factor ranging between 1.07-1.09.
Flue gas flowrate reassessment . Because the supplemental fuel combusted in CC is a part of the FG stream and fuel savings are achieved by WG preheating intensification, the amount of FG is reduced by the value of Δfs together with the corresponding amount of combustion air (as an oxidizer) calculated according to the oxidizer/fuel ratio K (see equation (5) below).Modified diagram plot . While the FG flowrate is corrected, the diagram of the existing unit ([Figure 2]) is modified as illustrated in [Figure 3]. By designed heat transfer intensification, the WG profile is extended by the value Qint, which causes a shift of the FG profile by the same value to the right. The FG gradient is also slightly increased due to the reduced flowrate.
From the above procedure described and [Figure 3], the FG high sensitivity to the ER modifications is obvious. This is an important aspect to be considered carefully during the conceptual design stage because it considerably influences the accuracy of the obtained results.
The FG profile shift causes a change of heat exchange driving forces in particular heat exchangers, thus it is recommended to re-evaluate the heat loads and to repeat the calculation procedure several times until the FG profile shift between iterations is reduced to a minimum.
The existing WG heater intensification
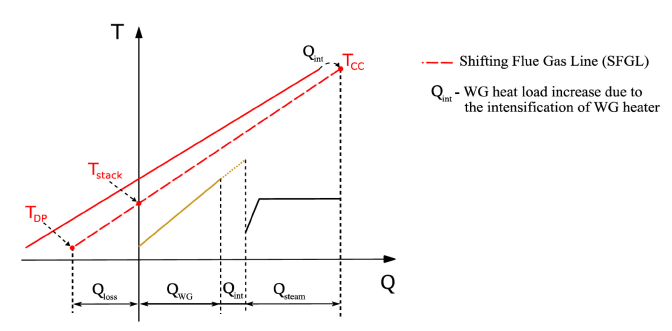
According to the energy balance of a combustion chamber, where the pollutant thermal decomposition takes place, the fuel savings could be reached by preheating any stream entering the CC. Besides the WG preheating, which is commonly employed (see [Figure 1]), another viable choice is, for example, preheating the combustion air (CA). If the WGtE unit processes several WG streams, while some of them are not preheated (mostly minor streams), the additional heat exchangers (preheaters) could be inserted in order to improve the FG heat utilization by preheating those streams.
The usual ER requirement is its minimal impact on the generation of energy media (e.g. steam generation illustrated in [Figure 1]). For this reason, new preheaters are recommended to be placed downstream to the energy media generators. A suitable position is commonly at the end of the FG flow path, where the waste heat (Qloss) could be directly utilized to reduce the current fuel demand without significant influence on other heat exchangers in HRS.
The insertion of a new preheater, however, influences the FG flowrate according to the same principle as in the case of the existing WG heater intensification discussed previously. The procedure of new preheater insertion consists of the following steps:
Exchanger minimum approach temperature (EMAT) value determination . EMAT evaluation is dependent on the existing HRS parameters and is discussed, for example, by Zhu and Asante [28].New preheater heat load calculation . According to the set EMAT value and known stream properties, the heat load (Qprh) and corresponding media (FG and, for example, CA) inlet/outlet temperatures are calculated.Fuel saving calculation and flue gas flowrate reassessment . Obtaining the fuel saving value Δfs and FG flowrate correction follows the same rules as in the case of the existing WG heater intensification.Modified diagram plot . A graphical representation of the SFGL method is performed according to slightly different rules than in the case of existing WG heater intensification. It is presented in the case of CA preheater insertion. When the FG flowrate is corrected, the modified diagram can be plotted as shown in [Figure 4]. The difference between the intensification of the existing preheater ([Figure 3]) and the insertion of the new one ([Figure 4]) is that the new preheater is placed to the left of the T-axis. This approach causes only the FG line rotation instead of shifting to the right as in the case of the existing preheater intensification.
The new preheater insertion
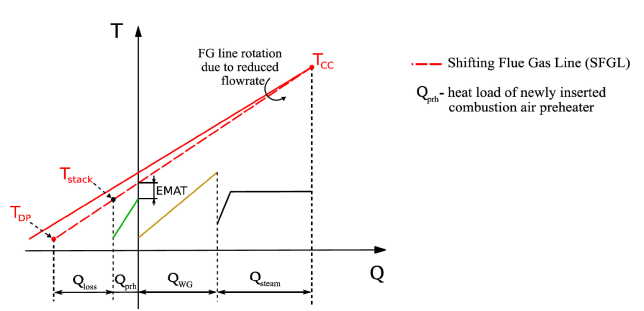
The rotation of the FG profile (as shown in [Figure 4]) causes breaking the CA preheater EMAT value in an initial iteration. The CA flowrate is also changed due to the reached fuel savings. For these reasons, it is necessary to perform several iterations of the procedure described above until the CA profile and FG profile (SFGL) are stable and the EMAT value requirement is fulfilled.
The ER performed by the insertion of a new preheater influences the heat exchange driving forces in existing heat exchangers in the same way as in the case of existing WG heater intensification described earlier. Thus the re-evaluation of heat loads in existing heat exchangers is recommended. However, if the FG flowrate change is small, the decrease in the heat transfer in existing heat exchangers could be neglected.
The developed SFGL method is further applied to a case study of a specific WGtE unit processing the WGs generated in an acrylic acid production plant.
The developed SFGL method here is practically introduced by its application to the ER design of a specific industrial WGtE unit, which serves thermal treatment of waste gas produced mainly from an acrylic acid producing process. The production process is a source of several waste gases containing VOC and CO in low concentrations (0.85 and 0.5 %vol). The current supplemental fuel consumption is required to be reduced by at least 30 % with as little modification of the current unit as possible. The paragraphs below introduce the studied WGtE unit with all key equipment.
The unit consists of a furnace, referred to as a combustion chamber (CC), where two WG streams are thermally treated – main waste gas (MWG) and secondary waste gas (SWG). The natural gas burner is employed in the furnace to promote thermal oxidation of the pollutants and thus to generate a flue gas (FG) at a high temperature (800 °C). FG waste heat is at first used to generate high pressure (HP) saturated steam, as a supplemental heating medium in the plant, and to superheat a medium pressure (MP) steam as a medium for power cycle (electricity generation).The MWG is then preheated in the main waste gas heater before entering the furnace (CC) to reduce the supplemental energy demand. The unit is illustrated in [Figure 5].
Studied WGtE unit
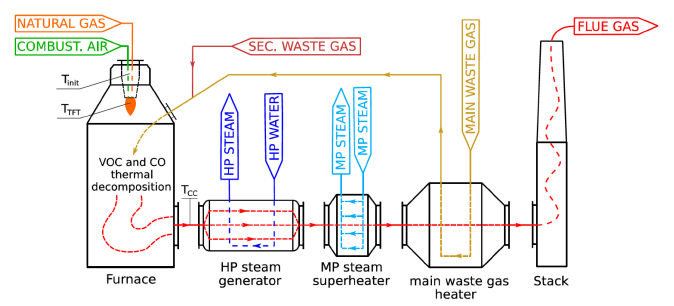
The current unit is energy very intensive, therefore the Energy Retrofit is requested in order to reduce the unit´s fuel consumption. The SFGL method was applied to design the technological modifications in the current HRS enabling it to reach the desired fuel saving by improving the FG heat recovery.
The unit´s HRS consists of three heat exchangers – HP steam generator, MP steam superheater, and MWG heater as shown in [Figure 5]. In the unit data extraction stage, their basic process and geometry characteristics were provided (see [Table 1]). According to the described SFGL procedure, the process stream characteristics are given in [Table 2] and fuel/oxidizer characteristics in [Table 3].
With the obtained data, the temperature-enthalpy diagram of the current unit was generated (see [Figure 6]). The FG line was extrapolated to the dew point temperature (TDP = 68.5 °C), which is according to the FG composition calculated as the temperature of water vapour condensation.
Existing heat exchangers´ process and geometry characteristics
Heat exchanger | Type | Hot side | Cold side | Heat load [kW] | ||
Fluid - location | Tin-out [°C] | Fluid - location | Tin-out [°C] | |||
HP generator | Plain tube, single pass | FG – tube side | 800-610 | HP – shell side | 100-211 | 1 702.0 |
MP superheater | Plain tube, multiple pass | FG – shell side | 610-460 | MP – tube side | 201-350 | 1 283.0 |
MWG heater | Plain tube, 2 pass | FG – shell side | 460-250 | MWG – tube side | 73-344 | 1 721.2 |
Basic characteristics of selected process streams
Stream | FG | MWG | SWG | HP | MP |
Flowrate [kg/h] | 23 279.2 | 18 158.6 | 2 364.6 | 2 602 | 13 000 |
Spec. heat capacity (cp)[kJ/(kg×K)] | 1.323 | 1.245 | 1.023 | – | 2.385 |
Fuel/oxidizer characteristics
Stream | Flowrate [kg/h] | LHV [MJ/kg] | FHVCC [MJ/kg] | cp [kJ/(kg×K)] | Tin [°C] | Tinit [°C] | TTFT [°C] | K [kg/kg] |
Natural gas | 130 | 49.08 | 29.95* | 2.206 | 20 | 42.56** | 1 805*** | 20.2 |
Combustion air | 2 626 | – | – | 1.012 | 45 |
Fuel Heating Value related to TCC (FHVCC) was calculated according to eq.(1), where nC= 1.07.
The initial fuel/oxidizer temperature (Tinit) is calculated according to gas mixture energy balance.
The Theoretical Flame Temperature (TTFT) is calculated as Adiabatic Flame Temperature.
Diagram of studied WGtE unit
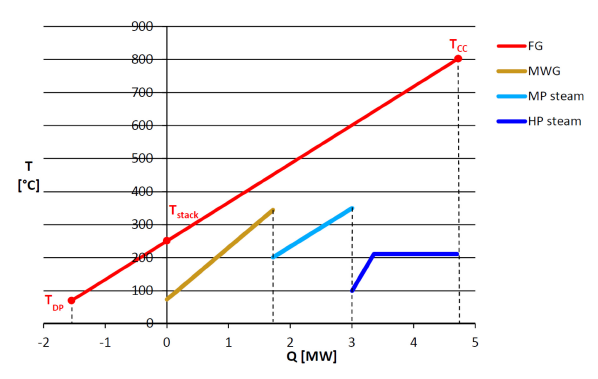
Approximate thermal efficiency of the current FG heat utilization was calculated according to equation (3) below. The thermal efficiency was related to the FG dew point (ηDP), which within the SFGL method represents the limit temperature to avoid FG condensation as mentioned in theMethods section and thus is also a limit point for the thermal efficiency calculation.
The amount of wasted heat (Qloss) was aproximately 1.553 MW. A part of this waste heat could be recovered in order to reduce the fuel consumption following the SFGL retrofit procedure.
(3)
To achieve the fuel savings, the heat enhancement of the MWG heater was performed first (according to the SFGL procedure). The analysis of the heat exchanger was carried out in the HTRI software, where the tube side was identified as the heat transfer controlling side (the side with a smaller heat transfer coefficient), which is suitable for heat enhancement.
Common techniques for tube side enhancement are internal fins, twisted-tape inserts, and coiled wire inserts. Coiled wire was chosen as an appropriate enhancement technique due to its low cost and considerable heat transfer increase with a reasonable rise in pressure drop. The heat duty of the MWG heater can be easily increased by 10% (170 kW).
The developed method was then applied for the fuel saving calculation and economical evaluation resulting from MWG heater enhancement. First, the fuel saving Δfs was calculated as shown in equation (4), where Qint was 170 kW and FHVCC was 29.95 MJ/kg (see [Table 3]). The combustion air demand reduction ΔmCA was obtained by air/fuel ratio K = 20.2 as performed in equation (5).
(4)
(5)
The fuel saving achieved by this minimal technological modification was 20.43 kg/h, which made a 15.72% fuel demand reduction in comparison with the current unit. According to the SFGL method presented in the Methods section, the flue gas flowrate, which was reduced by achieving the fuel saving, had to be reassessed as shown in eq. (6). The FG flowrate in the existing unit (mFG_exist= 23 279.2 kg/h, as shown in [Table 2]) was reduced by the proposed technological modification to the value 22 846 kg/h, which resulted in FG line shift in the temperature-enthalpy diagram as illustrated in [Figure 3].
(6)
The performed FG heat recovery enhancement reduced the heat loss, and also the FG stack temperature Tstack. In the existing unit, the stack temperature was 250°C (see [Table 1]). All necessary data for calculation of the stack temperature in the modified unit were known, as the FG specific heat capacity CP (see [Table 2]), the reassessed FG flowrate mFG_mod, and the heat load of the intensified MWG heater and other heat exchangers employed in the studied process (see [Table 1]), which were assumed to remain unchanged by the MWG heater intensification. The FG stack temperature in the modified unit was 219.2°C (i.e., 30.8 °C less than in the existing unit), which implied a considerable heat recovery improvement and thus the thermal efficiency increase, which rose from the current 75.2% (see eq. (3)) to 79.4%.
The annual financial benefit associated with the reached fuel saving could then be calculated. The unit annual operation period provided by the unit´s owner was 8 000 hours and the fuel cost was approx. 0.5 USD/kgfuel. The annual financial benefit resulting from the MWG heater intensification was then 81 570 USD.
The reached fuel saving notionally represents an equivalent of 340 tons of carbon dioxide (CO2) annual emission reduction. However, the required fuel saving was not met, so a new heat exchanger insertion was necessary as described below.
According to the SFGL method for ER of WGtE units, if enhancing the existing MWG heater is not possible or does not bring the desired fuel savings, then a new preheater must be employed (as described in the Methods section). In the studied WGtE unit ([Figure 5]), there were two streams available for this purpose – combustion air (CA) and secondary waste gas (SWG). The calculation procedure for a new heat exchanger introduction followed the procedure presented in Methods section and employed similar equations as in case of existing heat exchanger intensification as presented above in MWG heater intensification.
CA preheating is a commonly applied method to reduce the fuel consumption of process furnaces, industrial boilers, etc. In the studied WGtE unit, the CA stream was relatively small (see [Table 3]). Thus, the designed preheater was small enough to be introduced to the unit´s stack. The preheater´s EMAT value is estimated to be 40 °C, which guarantees efficient FG heat recovery along with a reasonably small size of the exchanger. The fuel saving calculation procedure was then applied according to the stated parameters for existing MWG heater intensification and CA preheater insertion. The achieved fuel saving was 22.67%, which was already a considerable fuel demand reduction, but it still did not meet the requirement, which was at least 30%. Increasing the CA preheater efficiency (by increasing heat transfer area or introducing enhancement techniques) could not bring the desired fuel savings either, because the preheater would reach its thermodynamic limit before the ER target is met.
As the intensification of the existing MWG heater together with the CA preheater introduction still did not meet the ER target, then another stream entering the furnace (CC) had to be preheated. The last available stream for this purpose was a secondary waste gas (SWG).
The SWG preheater was introduced to the stack downstream of the CA preheater. As the process characteristics of SWG were close to CA, the EMAT value of the SWG preheater was also estimated to be 40°C. As a result of the following SFGL application, the fuel saving reached by MWG heater intensification and two preheaters (CA and SWG) introduction was 30.35%, which met the ER target of the studied WGtE unit.
The specifications of proposed technological modifications are summarized in [Table 4] and the modified WGtEunit is further illustrated in [Figure 7]. A graphical illustration (diagram) of the performed SFGL method on the case study is illustrated in [Figure 8].
The payback period was only 5.5 months. This short payback period was achieved due to the low purchase cost of the new heat exchangers and pipelines. As the heat exchangers are standard tube bundles with no shell (as they are inserted into the chimney) and they operate under relatively low flue gas temperatures (around 200 °C), while flue gas condensation still does not occur, the heat exchangers and pipelines can be made from cheap carbon steel. Further, new pipelines were relatively short, as the whole WGtE unit covered a small built-up area. The cost for the purchase and installation of the heat exchangers, pipelines (including pipe insulation), and coiled wires (to MWG heater intensification) was estimated to be 60 130 USD. To cover any other possible costs, the expected purchase cost is increased by 20 %, i.e., 72 156 USD. Further, the operational costs of the modernized unit connected with the maintenance of newly added equipment were expected to be very negligible, because the new heat exchangers are small and easy to maintain and they could be cleaned along with the rest of the equipment (HP generator, MP superheater, and MWG heater).
The summarization and assessment of proposed technological modifications
Enhanced MWG heater | |
Applied enhancement technology | Coiled wire |
Increased heat transfer (Qint) | 170 kW |
Annual benefit | 81 570 USD |
CA preheater | |
Heat exchanger type | Plain tube, 2 pass, coiled wire inserts |
EMAT | 40 °C |
Heat transfer area | 31 m2 |
Heat duty | 64.8 kW |
Annual benefit | 30 945 USD |
SWG preheater | |
Heat exchanger type | Plain tube, 2 pass, coiled wire inserts |
EMAT | 40 °C |
Heat transfer area [m2] | 42 m2 |
Heat duty | 93.4 kW |
Annual benefit | 44 636 USD |
Fuel saving | 30.35% |
Approx. thermal efficiency (ηDP) | 83.43% |
Annual CO2 emission reduction | 853.8 tons |
Total annual benefit | 157 151 USD |
Expected payback | 5.5 months |
Modified WGtE unit
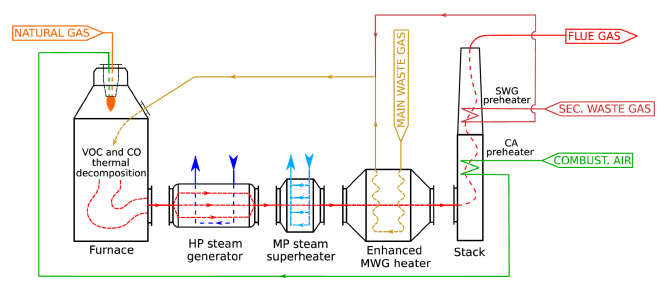
The SFGL diagram of studied WGtE unit
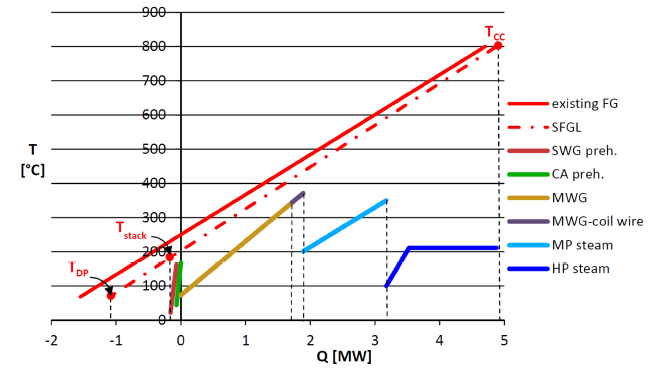
For a simple applicability of the SFGL method, a linear model of the studied process was applied. On the other hand, this simplification caused a reduced accuracy of obtained results. Several simplifications were also applied, such as neglecting the influence of technological modifications to the heat loads of the heat exchangers generating steam (HP generator and MP superheater).
The SFGL method’s accuracy and the validity of the established assumptions were verified by comparison of the obtained results with the non-linear simulation performed in software CHEMCAD, which was supported by advanced HTRI models of existing steam generators. The results of the performed comparison are summarized in [Table 5].
Comparison between the results obtained by SFGL method and non-linear simulation
Δfs[kg/h] | QHP [kW] | QMP [kW] | QMWG [kW] | QCA [kW] | QSWG [kW] | Qloss [kW] | |
SFGL method | 39.45 | 1 702.0 | 1 283.0 | 1 891.2 | 64.8 | 93.4 | 999.8 |
Non-linear simulation | 40.46 | 1 652.7 | 1 257.9 | 1 891.2 | 67.2 | 97.5 | 945.1 |
Deviation [%] | 2.5 | -3.0 | -2.0 | 0 | 3.6 | 4.2 | -5.8 |
The calculated fuel saving Δfs corresponds very well to the non-linear simulation results with a negligible difference of 2.5%. The supplemental fuel saving was thus calculated very accurately. Further, as FG flowrate was reduced along with achieved fuel savings, the heat loads of HP steam generator (QHP) and MP steam superheater (QMP) also slightly decreased. However, as the heat load reduction was not higher than 3%, it could be neglected and therefore the assumption of the constant heat load of the subject heat exchangers was a convenient calculation simplification.
The intensified heat transfer of the MWG heater (QMWG) by coiled wire insertion is highly dependent on the wire geometry. Therefore its heat load could be estimated as unchanged under slightly different operating conditions between the non-linear and SFGL model.
The heat loads of newly added CA and SWG preheaters (QCA and QSWG) also match very well. The differences (3.6 % and 4.2%) are caused by inevitable inaccuracies during the data gathering stage, especially the streams´ predicted cp values.
The obtained value of wasted heat (Qloss) also shows a very good match, while the difference (5.8%) is caused by linear extrapolation between Tstack and TDP, which does not follow the real FG profile accurately. However, in the conceptual design stage of the unit Energy Retrofit, the obtained results are fairly accurate.
In this paper, a systematic analytical method for efficient and fairly accurate conceptual design for Energy Retrofit of WGtE units has been proposed. A linear model of flue gas profile varying during the ER procedure is introduced – the Shifting Flue Gas Line (SFGL). The presented method provides the initial analysis in terms of utilizable waste heat contained in the FG stream produced in the WGtE unit and it provides the basic technological modification design and fuel saving assessment. It applies the temperature enthalpy linear model of the unit´s process streams together with its graphical representation to promote interactivity during the design procedure. The developed method was further applied to a case study of an industrial WGtE unit processing a waste gas containing VOC and CO. By strategic technological modifications, considerable fuel savings (over 30%) were achieved with a payback period of less than 6 months. The results´ accuracy was further confirmed by comparing it to an advanced non-linear simulation of the studied process.
In summary, the proposed SFGL method represents a straightforward, systematic, and easily applicable conceptual design tool for performing the ER of WGtE units. The method is for a specific application. It is therefore not recommended to be applied beyond the WGtE technology.
Symbols | ||
CO | carbon monoxide | |
CO2 | carbon dioxide | |
cp | specific heat capacity | [kJ/(kg×K)] |
FHVCC | Fuel Heating Value utilizable to heat the combustion chamber | [MJ/kg] |
K | oxidizer/fuel ratio | [kg/kg] |
mFG_exist | flue gas flowrate in existing waste gas-to-energy unit | [kg/h] |
mFG_mod | flue gas flowrate in modified (enhanced) unit | [kg/h] |
nc | correction factor | [-] |
Q | heat load | [kW] |
QCA | combustion air preheater heat load | [kW] |
QHP | heat load for high-pressure steam generation | [kW] |
Qint | heat load increase (intensification) | [kW] |
Qloss | heat loss | [kW] |
QMP | heat load for medium-pressure steam generation | [kW] |
QMWG | heat load for main waste gas heating | [kW] |
Qprh | heat load of newly inserted preheater | [kW] |
Qsteam | heat load for steam generation | [kW] |
QSWG | heat load for secondary waste gas preheating | [kW] |
QWG | heat load for waste gas heating | [kW] |
T | temperature | [°C] |
TCC | flue gas temperature at the outlet of combustion chamber | [°C] |
TDP | flue gas dew point temperature | [°C] |
Tin | inlet temperature | [°C] |
Tinit | initial temperature of oxidizer/fuel mixture | [°C] |
Tout | outlet temperature | [°C] |
Tstack | flue gas stack temperature | [°C] |
TTFT | Theoretical Flame Temperature | [°C] |
Δfs | fuel saving | [kg/h] |
ΔmCA | combustion air flowrate reduction | [kg/h] |
ηDP | flue gas utilization thermal efficiency related to dew point, | [%] |
Abbreviations | |
CA | combustion air |
CC | combustion chamber |
EMAT | exchanger minimum approach temperature |
ER | Energy Retrofit |
FG | flue gas |
HEN | Heat Exchanger Network |
HP | high-pressure (steam) |
HRS | Heat Recovery System |
LHV | Lower Heating Value |
MP | medium-pressure (steam) |
MWG | main waste gas |
SFGL | Shifting Flue Gas Line |
SWG | secondary waste gas |
VOC | Volatile Organic Compound |
WG | waste gas |
WGtE | Waste Gas-to-Energy (unit) |
- , , Air Pollution Control Technology Handbook, 2015
- , , Sustainable Process Integration and Intensification: Saving Energy, Water and Resources, 2018
Synthesis of heat exchanger networks: I. Systematic generation of energy optimal networks ,AIChE Journal , Vol. 24 (4),pp 633-642 , 1978, https://doi.org/https://doi.org/10.1002/aic.690240411
, Cost-effective strategy for heat exchanger network retrofit ,Energy , Vol. 146 ,pp 82-97 , 2018, https://doi.org/https://doi.org/10.1016/j.energy.2017.09.005
, A novel heat exchanger network retrofit approach based on performance reassessment ,Energy Conversion and Management , Vol. 177 ,pp 477-492 , 2018, https://doi.org/https://doi.org/10.1016/j.enconman.2018.10.001
, Heat Exchanger Network Retrofit Considering Physical Distance, Pressure Drop and Available Equipment Space ,Chemical Engineering Transactions , Vol. 76 ,pp 367-372 , 2019, https://doi.org/https://doi.org/10.3303/CET1976062
, A Steam Utility Network Model for the Evaluation of Heat Integration Retrofits - A Case Study of an Oil Refinery ,Journal of Sustainable Development of Energy, Water and Environment Systems , Vol. 5 (4),pp 560-578 , 2017, https://doi.org/https://doi.org/10.13044/j.sdewes.d5.0167
, Heat transfer enhancement, intensification and optimisation in heat exchanger network retrofit and operation ,Renewable and Sustainable Energy Reviews , Vol. 120 ,pp 109644 , 2020, https://doi.org/https://doi.org/10.1016/j.rser.2019.109644
, The importance of thermodynamic insight in Work and Heat Exchange Network Design , Vol. 81 ,pp 127-132 , 2020, https://doi.org/https://doi.org/10.3303/CET2081022
, Integration of energy recovery network including recycling residual pressure energy with pinch technology ,Chinese Journal of Chemical Engineering , Vol. 25 (4),pp 453-462 , 2017, https://doi.org/https://doi.org/10.1016/j.cjche.2016.07.020
, Work Exchange Networks (WENs) and Work and Heat Exchange Networks (WHENs): A Review of the Current State of the Art ,Ind. Eng. Chem. Res , Vol. 59 (2),pp 507-525 , 2020, https://doi.org/https://doi.org/10.1021/acs.iecr.9b04932
, A methodology for designing thermodynamic energy conversion systems in industrial mass/heat integration problems based on MILP models ,Energy , Vol. 185 ,pp 121-135 , 2019, https://doi.org/https://doi.org/10.1016/j.energy.2019.06.124
, Two-step MILP/MINLP approach for the synthesis of large-scale HENs ,Chemical Engineering Science , Vol. 197 ,pp 432-448 , 2019, https://doi.org/https://doi.org/10.1016/j.ces.2018.06.036
, Synthesis and optimization of work and heat exchange networks using an MINLP model with a reduced number of decision variables ,Applied Energy , Vol. 262 ,pp 114441 , 2020, https://doi.org/https://doi.org/10.1016/j.apenergy.2019.114441
, Retrofitting of municipal coal fired heating plant with integrated biomass gasification gas turbine based cogeneration block ,Energy Conversion and Management , Vol. 51 (5),pp 1085-1092 , 2010, https://doi.org/https://doi.org/10.1016/j.enconman.2009.12.014
, Energy and economic optimization of the repowering of coal-fired municipal district heating source by a gas turbine ,Energy Conversion and Management , Vol. 149 ,pp 885-895 , 2017, https://doi.org/https://doi.org/10.1016/j.enconman.2017.03.053
, Tri-generation System based on Municipal Waste Gasification, Fuel Cell and an Absorption Chiller ,Journal of Sustainable Development of Energy, Water and Environment Systems , Vol. 6 (1),pp 13-32 , 2018, https://doi.org/https://doi.org/10.13044/j.sdewes.d5.0172
, An Integrated Approach for Analysis of Higher Penetration of Variable Renewable Energy: Coupling of the Long-Term Energy Planning Tools and Power Transmission Network Models ,Journal of Sustainable Development of Energy, Water and Environment Systems , Vol. 7 (4),pp 615-630 , 2019, https://doi.org/https://doi.org/10.13044/j.sdewes.d7.0264
, Stability Enhancement of a Power System Containing High-Penetration Intermittent Renewable Generation ,Journal of Sustainable Development of Energy, Water and Environment Systems , Vol. 3 (2),pp 151-162 , 2015, https://doi.org/https://doi.org/10.13044/j.sdewes.2015.03.0012
, Practical Energy Retrofit of Heat Exchanger Network Not Containing Utility Path ,Energies , Vol. 13 (11),pp 2711 , 2020, https://doi.org/https://doi.org/10.3390/en13112711
, Innovative Method for Fuel Saving Calculation Related to Energy Retrofit of Thermal Waste Processing Units ,in Proceedings of the Engineering Mechanics 2020, Svratka, Czech Republic , Vol. 26 ,pp 142-145 , 2020, https://doi.org/https://doi.org/10.21495/5896-3-142
, - Chemstations, 2018, https://www.chemstations.com/
- HTRI | HTRI, https://www.htri.net/
Appropriate placement of Furnaces in the Integrated Process ,presented at the IChem Symposium “Understanding Process Integration II”, UMIST, Manchester , 1988
, Furnace integration into processes justified by detailed calculation using a simple mathematical model ,Chemical Engineering and Processing: Process Intensification , Vol. 34 (1),pp 9-23 , 1995, https://doi.org/https://doi.org/10.1016/0255-2701(94)00508-7
, Plant energy saving through efficient retrofit of furnaces ,Applied Thermal Engineering , Vol. 20 (15),pp 1545-1560 , 2000, https://doi.org/https://doi.org/10.1016/S1359-4311(00)00031-4
, Study on the Potential of an Integrated Waste-to-Energy Unit ,M.Sc Thesis, Brno University of Technology, Brno, Czech Republic , 2019
, Diagnosis and optimization approach for heat exchanger network retrofit ,AIChE Journal , Vol. 45 (7),pp 1488-1503 , 1999, https://doi.org/https://doi.org/10.1002/aic.690450712
,