Oilfield-produced water (OPW) represents the most significant wastewater stream in oil exploration and production processes. As the demand for water by industries worldwide continues to grow, developing strategies to optimise water use and recovery will become increasingly critical in the coming decades [1]. However, the treatment of OPW is not trivial, usually including physical, chemical, and biological steps to meet acceptable legal requirements for discharge, making the development of efficient wastewater treatment technologies for OPW a major challenge for the oil industry [2]. Such treatments [3] address economic sustainability but raise concerns about environmental sustainability. Unfortunately, this aspect is frequently overlooked in the context of remediation activities, although they are intended to reduce the overall footprint of human activity.
However, remediation activities also have an impact on the environment because the products or chemical processes involved may consume materials and energy [4] or lead to the unwanted production of toxic byproducts (secondary contamination) [5]. It can severely affect the sustainability of the remediation intervention itself [6]. An environmentally friendly and sustainable alternative is represented by remediation technologies based on natural solutions, central among which is phytoremediation [7], an approach that uses plant species to address organic and inorganic contaminants in the target matrix by synergistically exploiting a variety of mechanisms such as extraction [8] or degradation [9].
The low cost and ease of operation, combined with the demonstrated environmental benefits [10], are fueling interest in these phytotechnologies, including in combination with other green strategies to further reduce the overall carbon footprint, such as treatment trains [11] or energy recovery from light radiation [12]. Also included in this discourse are green roofs [13] and green-based materials derived from agricultural wastes [14], which enable improved energy and environmental performance of urbanised areas by combining constructed wetland techniques with other approaches to alleviate the growing effects of climate change [15]. In particular, constructed wetlands (CWs) [16] could be an alternative method to treat OPW economically and sustainably compared to conventional methods [17]. However, OPW is also characterised by significant salinity values. Therefore, selecting halophilic species is necessary to develop an effective phytoremediation system.
The progressive increase in the desertification process caused by climate change extends hypersaline environments worldwide [18]. Rising global temperatures and precipitation variability favour wet and dry deposition of sea salt (NaCl) from precipitation and wind fluxes by stimulating the release of salts from physicochemical rock erosion. However, extreme salinity levels can also be caused by poor soil management, excessive use of fertilisers, irrigation with saline-sodic wastewater, and surface or groundwater seawater intrusion. This salinisation increase and freshwater availability decrease negatively affect crop productivity, drastically reducing arable land [19]. Seawater intrusion due to groundwater overexploitation is widely found in the coastal areas of the Italian, Spanish and Greek peninsulas and North African regions [20]. Furthermore, large coastal regions of the Arabian Gulf countries are hypersaline due to using open basins to discharge treated OPW [21]. Although the presence of trace hydrocarbons does not represent a serious problem, high salinity, with average ranges of 1–250 g L-1, is a major problem in OPW management [22]. Indeed, such high levels of NaCl are unfavourable for the growth of most plants and microorganisms. Therefore, this may represent a limiting factor for using phytoremediation to treat OPW [23]. For phyto-desalination (saline phytoremediation), halophyte species are needed, representing only 1% of the world's flora.
Numerous studies have been conducted on the salinity tolerance of halophytes, and the biochemical mechanisms implemented by plants to counteract salt stress have also been investigated [24]. However, most of these studies are limited to salt concentrations typical of seawater (35 g L-1) under hydroponic culture conditions [25]. Halophilic species such as Phragmites australis and Thypa latifolia have been tested to treat relatively low salinity water production (12 g L-1) with vertical-flow CWs. P. australis was less sensitive than T. latifolia to salt concentration, effectively removing benzotriazole and benzoic acid as additives in the treated water [26]. However, most produced waters have much higher saline levels, and it is therefore essential to evaluate the ability of plants to survive and tolerate the specific saline conditions of the wastewater. In the presence of high salt concentrations, plants' first defence mechanisms are the closure of the stomata and the inhibition of photosynthesis, reducing growth and creating severe dehydration [27]. Plant-associated microorganisms play an essential role in the evolutionary adaptation of plants to climatic stresses such as drought and salinity. Rhizospheric microorganisms and endophytes can mitigate the negative effects of water shortage and excess salt through various mechanisms, such as the solubilisation of nutrients and the production of phytohormones and exopolysaccharides [28]. Therefore, the first screening of the hypersaline response in halophytes must consider these physiological and chemical characteristics.
Some plants that colonise the humid areas typical of estuaries have been selected for the first tolerance tests to extreme saline conditions. Estuaries are bodies of water that connect the land with the sea, where the influx of freshwater dilutes the seawater. Among the species selected for testing were Juncus maritimus Lam., Phragmites australis (Cav.) Trin. ex Steud., Halocnemum strobilaceum (Pallas) M. Bieb. and Suaeda fruticosa (L.) Forsk. Following this first tolerance analysis, the two best species were chosen for experimentation in mesocosms with increasing concentrations of salts and hydrocarbons to evaluate their growth in hypersaline conditions and their potential hydrocarbon degradation activity.
Below are details on the experiments, from plant selection to mesocosm test setup.
Young seedlings were used to evaluate tolerance to increasing concentrations of NaCl by performing vegetative propagation with organic soil. Semi-control conditions were adopted for plant growth conducted in a greenhouse [29]. For subsequent transfer to hydroponic conditions, the soil was carefully removed from the roots, and the seedlings were then placed in plastic pots containing 3 litres of hydroponic solution. The hydroponic solution was prepared with a commercial complete liquid fertiliser, COMPO Italia s.r.l., diluted in tap water.
After verifying by visual symptoms of adequate acclimation to hydroponic conditions, the four plants were subjected to NaCl concentrations 15, 30, 50 and 100 g L-1 (257, 514, 856 and 1712 mM NaCl), respectively, for 28 days. NaCl was added gradually to avoid osmotic shock, with a daily application (2.5−5 g L-1 NaCl) of salt until the desired concentration was reached. For each species and salt treatment, two mesocosms were used with two plants of the same species for 40 mesocosms. The hydroponic solution (pH = 5) was replaced every 7 days, keeping the established salt and pH conditions unchanged to minimise any changes in osmotic potential.
Chlorophyll fluorescence measurements were performed to assess the effect of treatments on photosystem II (PSII) functionality. The actual photochemical efficiency of PSII in the light (ΦPSII) was measured under growing light conditions (~100 mmol photons m-2 s-1) on leaves of each experimental plant using a pulse-amplitude-modulated fluorometer (Mini-PAM; Heinz Walz GmbH, Effeltrich, Germany).
Plant biomass as dry weight was evaluated at the end of the test by drying the harvested plants in an oven at 60 °C until a constant weight was reached.
The adopted methodology for the hydrocarbon degradation test was the "hydroponic mesocosm", i.e., pots equipped with a specific water re-circulation system to assure optimal plant growth, maximising the interaction of the oil phase with plant roots. The experimental campaign was performed in a greenhouse (Figure 1), adopting the same conditions of temperature, relative humidity (RH), photosynthetic active radiation (PAR), and CO2 concentration given in the previous section.
Picture of the experimentation in mesocosm carried out in the greenhouse
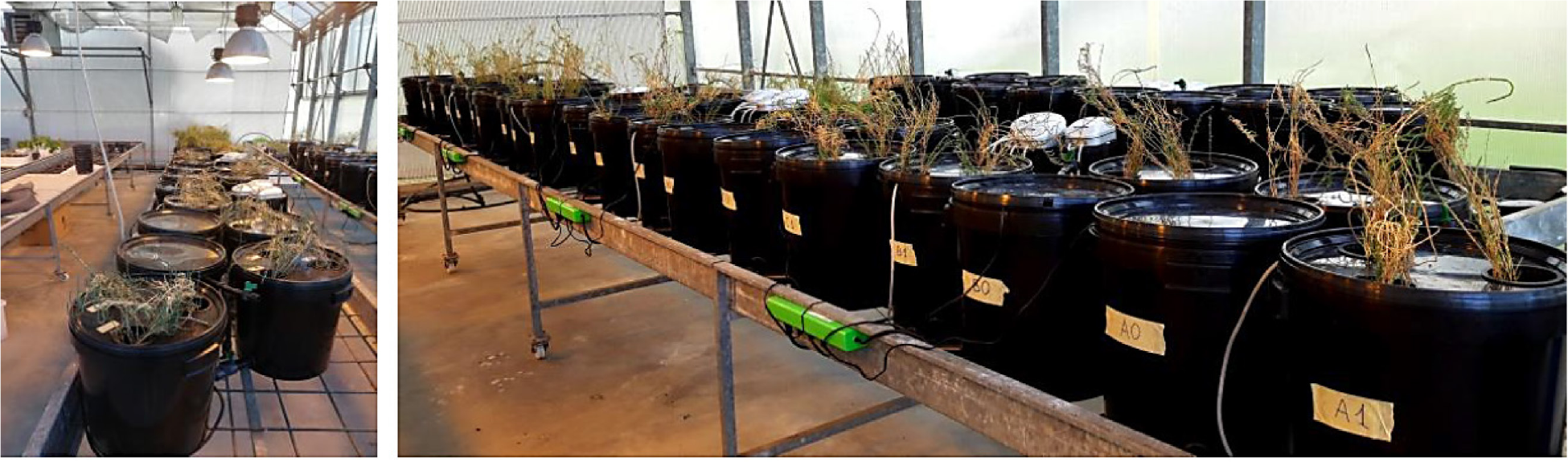
The test involved the installation of 12 hydroponic systems, which consisted of three pots of 28 L each (36 cm diameter and 38 cm height), connected by plastic tubes. Two pots of the hydroponic system were dedicated to plant cultivation. The third pot was equipped with mechanical pumps to ensure the circulation of the solution in all three pots. An air pump was connected with a tube, and an air stone lay at each pot's bottom to ensure aeration. This hydroponic system guarantees the best-growing conditions for the plants for a long-lasting experiment, avoiding the repeated change of the nutrient solution but allowing fertiliser addition when necessary.
The nutrient solution pH was monitored throughout the experiment with a portable pH meter and kept constant at a value of 5 by adding the required doses of KOH (1 N) as, for an extended period, the hydroponic solution tends to decrease the pH, probably due to the effects of the rhizosphere activity. All plants were firstly acclimatised to the greenhouse environment for about 10 days and then properly adapted and transferred to hydroponic growth systems.
Three different salt concentrations were tested (0, 50, 65 g L-1) with 600 mg kg-1 oil (petroleum hydrocarbons) added. The experimentation lasted 18 months, and for each plant species, six treatments were set up (Table 1) with a unique application of oil, i.e., without replenishment.
Mesocosms treatments description: the basis of all tests in control (CT) and synthetic water (SW) pots is a nutrient solution at pH = 5
Sample | Salt [g L-1] | Petroleum Hydrocarbons [mg kg-1] | Aeration |
---|---|---|---|
CT | --- | --- | Yes |
SW50 | 50 | --- | Yes |
SWH | --- | 600 | Yes |
SW50H | 50 | 600 | Yes |
SW50HnoA | 50 | 600 | No |
SW65H | 65 | 600 | Yes |
At the end of the experimental trial (18 months), plants were collected and oven-dried at 60 °C to determine the dry weight of the total biomass. Total Petroleum Hydrocarbons (TPH) and hydrocarbon speciation analyses were performed on water samples. The TPH content was determined according to EPA 5021A 2014 + EPA 3510C 1996 + EPA 8015C 2007, and hydrocarbon speciation was performed according to the Massachusetts Department of Environmental Protection (MADEP) methodology [30], [31], while the EPA 3510C 1996 + EPA 8270E 2018 protocol was used for polycyclic aromatic hydrocarbons (PAHs) [32], [33].
Root samples exposed to different salt and hydrocarbon treatments were examined for the metagenomic analysis of the endophytic microbial communities. Specifically, when plants were taken from the mesocosms, the root portions for metagenomic analysis were immediately processed for DNA extraction and then subjected to metagenomic sequencing, as reported by Franchi et al. [9].
The bacterial strains were then characterised to determine their possession of plant growth-promoting (PGP) properties.
As mentioned, the salt tolerance tests involved four different plant species. All species have adapted to growth in hydroponic culture but show different resistance to salt stress (0−15−30−50−100 g L-1). Resistance to salt stress was assessed by measuring the actual photochemical efficiency of photosystem II in the light (pPSII). This analysis showed that up to 50 g L-1 of NaCl, the species H. strobilaceum and S. fruticosa showed a slight decrease in PSII. On the other hand, the highest dose (100 g L-1) caused a strong decrease in photochemical efficiency. In contrast, in J. maritimus and P. australis, the negative effect of salt on photosystem II started at 30 g L-1, and at 50 g L-1, PSII was close to zero, as previously reported [29].
This initial tolerance analysis identified H. strobilaceum and S. fruticosa as the best species for growing under hypersaline conditions. Hence, these species were selected to test their potential hydrocarbon degrading activity in the mesocosm experiment. Accordingly, the PSII of H. strobilaceum and S. fruticosa plants growing in the mesocosm was only slightly affected by the addition of salt (50 g L-1 NaCl), especially H. strobilaceum, which showed values of photochemical efficiency and aboveground biomass that were not significantly different from the control (Table 2).
Actual photochemical efficiency of PSII (.PSII) and aboveground biomass of H. strobilaceum and S. fruticosa growing in mesocosms (CT, control; SW50, synthetic water with 50 g L-1 of NaCl)
Sample | H. strobilaceum | S. fruticosa | ||
---|---|---|---|---|
ΦPSII | Biomass per pot [g] | ΦPSII | Biomass per pot [g] | |
CT | 0.728 ± 0.009 | 24.5 ± 2.5 | 0.734 ± 0.006 | 33.5 ± 5.6 |
SW50 | 0.708 ± 0.027 | 22.3 ± 1.2 | 0.631 ± 0.022 | 6.7 ± 1.8 |
The results obtained from such mesocosm experiments reveal a species-specific tolerance to hypersaline conditions and the degradation of crude oil hydrocarbons for the two selected plants. H. strobilaceum showed better tolerance to both salinity and hydrocarbons, but S. fruticosa showed the best performance in hydrocarbon biodegradation.
After eight months from the oil addition, each sample showed a much lower total petroleum hydrocarbons (TPH) content than hydrocarbon added (T0). Moreover, vegetated mesocosms showed different TPH contents and speciation patterns depending on the plant species, salt treatment, and aeration (Figure 2).
The analysis of the hydrocarbon chain speciation (Figure 3) showed that after eight months, the residual TPH fraction was composed only of aliphatic chains C19−C36 and C13−C18 in vegetated and non-vegetated mesocosms. The fraction of polycyclic hydrocarbons (PAHs) seems to have been completely degraded in the mesocosms with S. fruticosa. At the same time, some PAH remains, even if in small quantities, in the non-aerated mesocosms (SW50HnoA) with H. strobilaceum (Figure 3).
Comparison of the TPH content detected in the waters of the mesocosms with the two species S. fruticosa and H. strobilaceum after eight months of experimentation (CT, control; SW, synthetic water; NV, non-vegetated; noA, non-aerated)
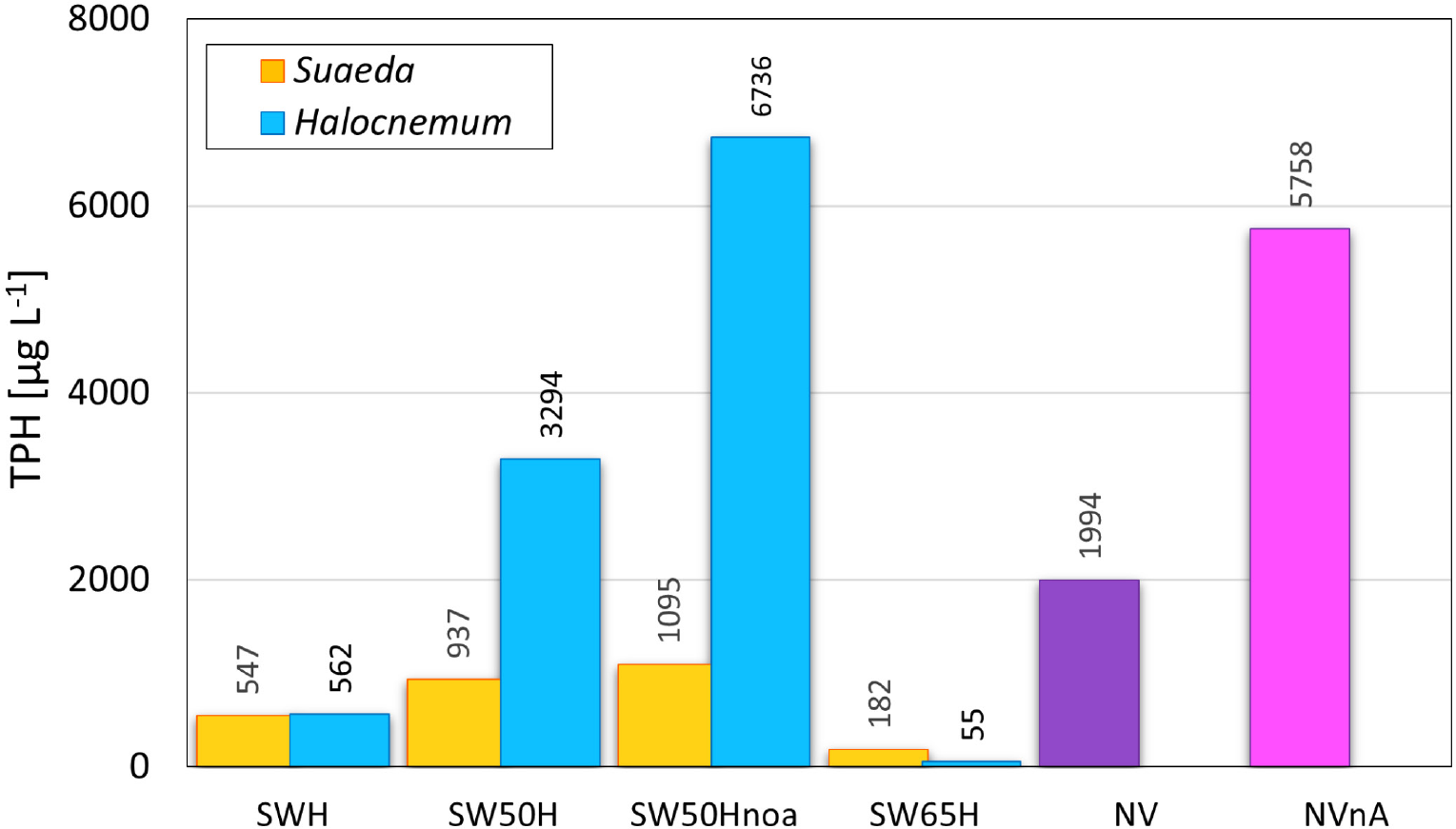
Data of gas chromatography – mass spectrometry analysis from the water sampled at the end of the mesocosm experiment
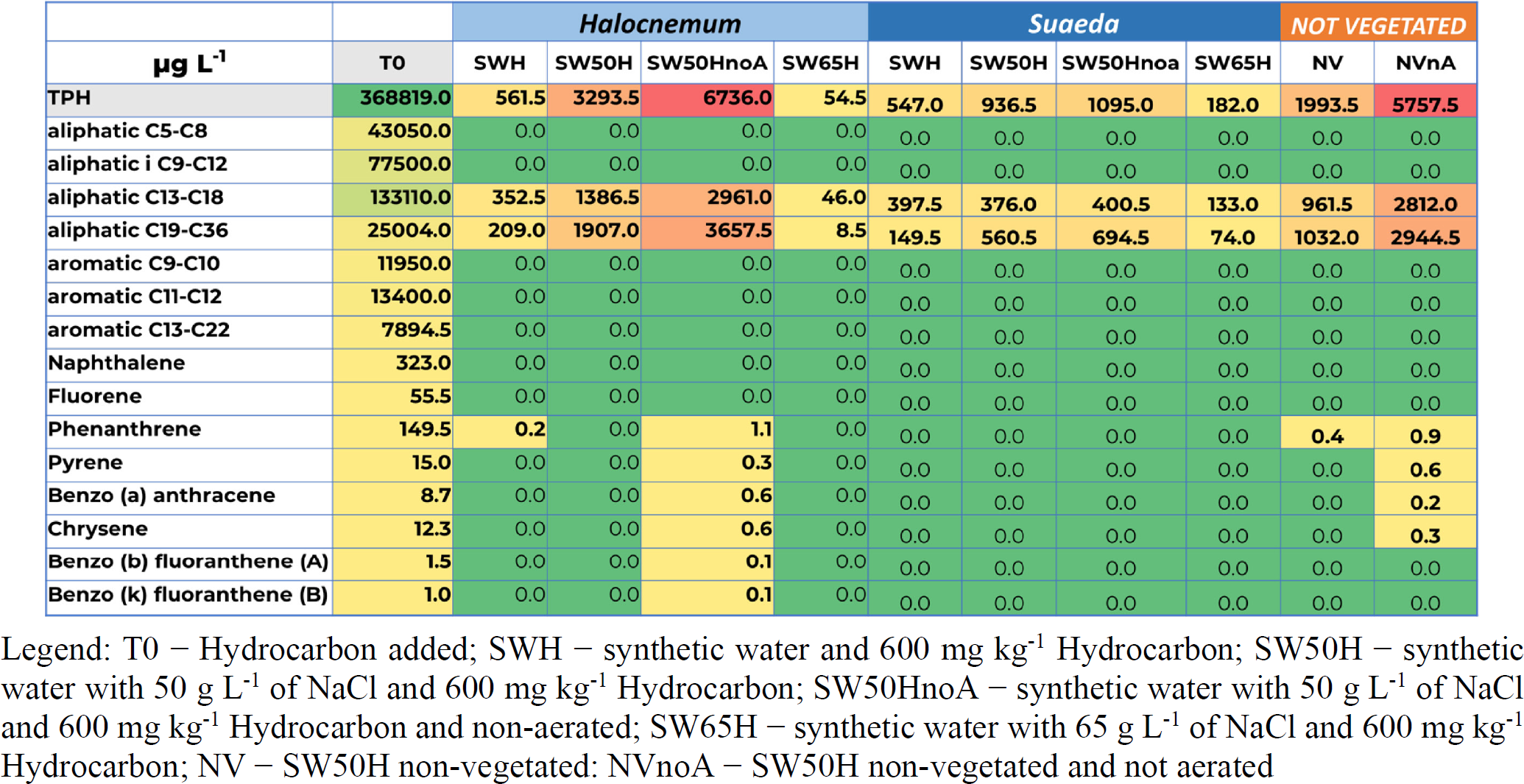
Figure 3 also notes how the high saline content can affect microbial growth and activity; the mesocosms with the highest salt content (65 g L-1) showed the lowest TPH content.
At the end of the experiment, a metagenomic analysis was performed of the endophytes related to the roots of the samples coming from the three SW treatments, SW50H50, SWH50noA, and SW65H (Figure 4).
In Figure 4, metagenomic information is expressed as a relative abundance percentage. Therefore, the greater the presence of a particular group of microorganisms, the more significant the percentage shown in the histogram.
Metagenomic analysis of the roots from S. fruticosa and H. strobilaceum at the end of the trial; the graph shows that the different experimental conditions led to significant family speciation
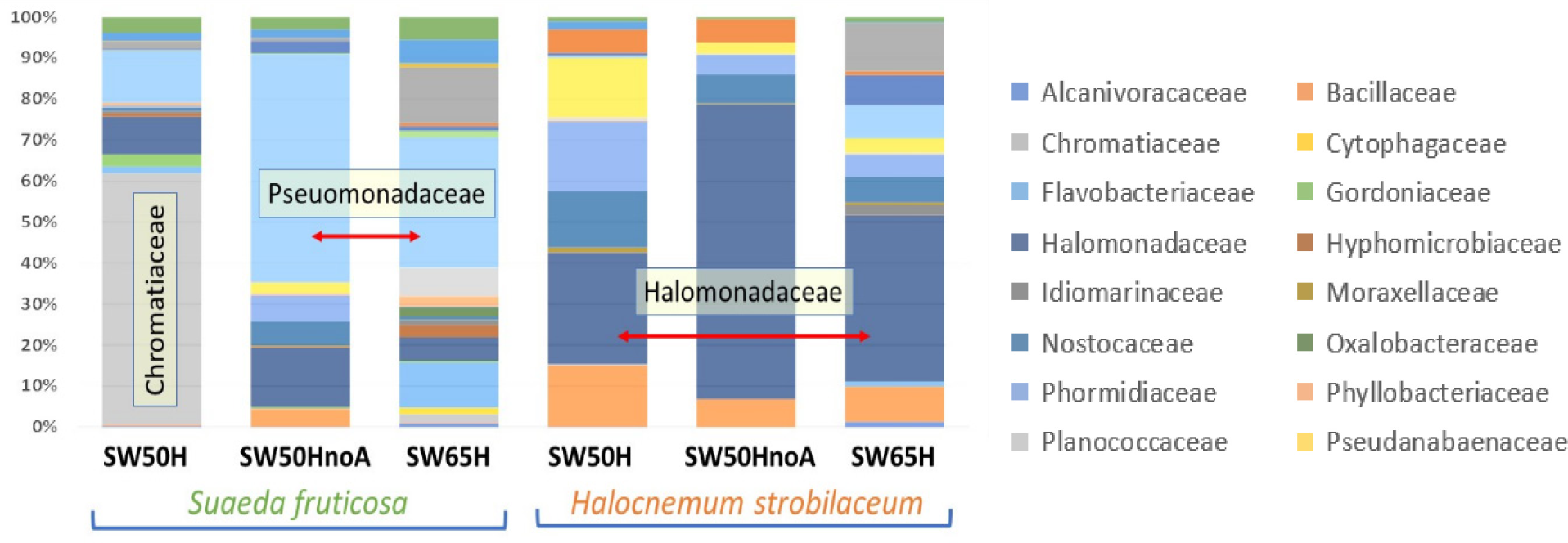
The metagenomics results confirmed the specificity of the two plants by showing significant speciation in the detected microbial communities, especially at the family level, which proved to be a more meaningful comparison than Phylum or Genera (a greater difference in the percentage of occurrence in the different samples analysed). The Pseudomonadaceae family appears to prevail in mesocosms with S. fruticosa, while the Halomonadaceae family is more abundant in those with H. strobilaceum (Figure 4). Halophytes are naturally salt-tolerant plants that have evolved to grow in saline soils, and this evolution is coupled with the selection of the rhizosphere microorganisms associated with their roots. However, while the plant physiology of these plants, and in particular the mechanisms involved in salt tolerance, have been studied extensively, little is known about the potential contribution of the rhizobacteria associated with these plants, particularly the endophytes, which can contribute significantly to mitigating adverse conditions and thus supporting plant growth. In this regard, endophytes associated with mesocosms were also studied in the present experiment. Specifically, the endophytes isolated and characterised for their growth-promoting properties are shown in Figure 5.
Isolated endophytes and related in vitro plant growth-promoting; the + sign indicates the presence of the PGP property, and the − sign its absence; each strain was assigned a score calculated considering the number of PGP properties displayed
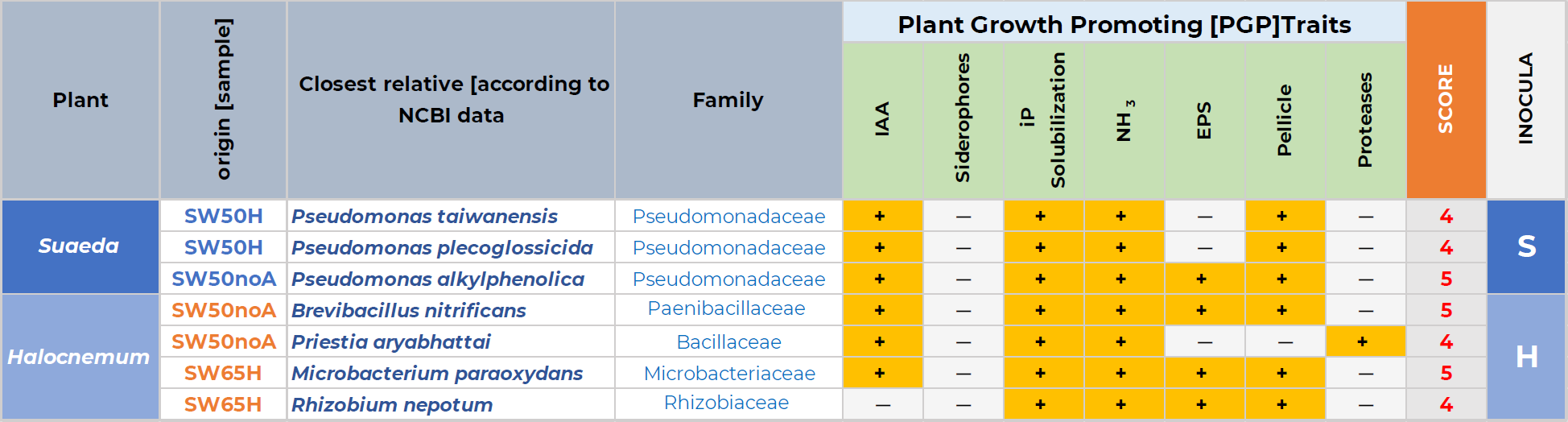
The effect of selected endophytes on biomass growth, photochemical efficiency, and general stress resistance requires a new ad hoc experimental campaign, which will be the subject of future contributions.
Phytoremediation is an in-situ technology that is intrinsically applicable over large areas. However, salinity usually places severe limitations on using plants as a means of remediation.
The preliminary activity described concerns identifying and selecting halophilic species potentially suitable for growing/surviving in evaporation ponds where the water used for oil extraction is only partially collected, as most of it is reinjected into the wells.
The halophilic species H. strobilaceum and S. fruticosa, selected from previous research, were subjected to mesocosm experiments with the simultaneous introduction of salt and oil. The results revealed distinct responses to saline conditions and different abilities to degrade the hydrocarbons present in the crude oil sample. Consistent with previous findings, H. strobilaceum demonstrated greater resilience to hypersalinity, with minimal effects on photosynthetic efficiency and only a slight decrease in biomass. Conversely, S. fruticosa experienced a significant reduction in biomass and a slight decrease in photosynthetic efficiency. At the same time, hydrocarbon analysis indicated that mesocosms containing S. fruticosa outperformed those with H. strobilaceum in hydrocarbon reduction.
Metagenomic analysis of plant root-associated microbial communities shows significant and interesting differences between experimental conditions and between the two species. Further studies would test the effect of adding isolated endophytic bacteria on improving plants' resistance to hypersalinity and hydrocarbon reduction.
It is, therefore, a system that is technically applicable to real conditions and can be easily implemented, for example, in terrestrial areas where space is not a limiting factor and where evaporation ponds already exist. The possibility of applying an approach such as phytoremediation under the above conditions is an environmentally sustainable and desirable option, and this investigation allowed us to define an area where the technology could be used as an alternative to the consolidated technologies for treating produced water.
The research was supported and fully funded by Eni S.p.A. (contract N. 3500047415, within the framework agreement N. 4400003660). The authors thank Irene Rosellini, Manuele Scatena, Annalisa Meucci, and Valerio Lazzeri (IRET-CNR) for technical assistance.
Abbreviations | |
---|---|
CT | Control |
CW | Constructed Wetland |
PAH | Polycyclic Aromatic Hydrocarbon |
PGP | Plant Growth Promoting |
PS | Photosystem |
PS | Photochemical Efficiency of Photosystem |
SW | Synthetic Water |
TPH | Total Petroleum Hydrocarbons |
Improved management of water resources in process industry by accounting for fluctuations of water content in feed streams and products ,Journal of Water Process Engineering , Vol. 39 ,pp 101870 , 2021, https://doi.org/https://doi.org/10.1016/j.jwpe.2020.101870
, Review of oilfield produced water treatment technologies ,Chemosphere , Vol. 298 ,pp 134064 , 2022, https://doi.org/https://doi.org/10.1016/j.chemosphere.2022.134064
, Evaluation of the environmental and economic performance of wastewater treatment technologies of warm climate regions ,Journal of Sustainable Development of Energy, Water and Environment Systems , Vol. 11 (4),pp 1–17 , 2023, https://doi.org/https://doi.org/10.13044/j.sdewes.d11.0472
, Green Synthesis of Silver Nanoparticles by Low-Energy Wet Bead Milling of Metal Spheres ,Materials , Vol. 13 (1),pp 63 , 2019, https://doi.org/https://doi.org/10.3390/ma13010063
, Removal and recovery of heavy metals from tannery sludge subjected to plasma pyro-gasification process ,J Clean Prod , Vol. 273 ,pp 123166 , 2020, https://doi.org/https://doi.org/10.1016/j.jclepro.2020.123166
, Sustainability in ElectroKinetic Remediation Processes: A Critical Analysis ,Sustainability , Vol. 13 (2),pp 770 , 2021, https://doi.org/https://doi.org/10.3390/su13020770
, Soil Remediation: Towards a Resilient and Adaptive Approach to Deal with the Ever-Changing Environmental Challenges ,Environments , Vol. 9 (2),pp 18 , 2022, https://doi.org/https://doi.org/10.3390/environments9020018
, Applicability of a Freundlich-Like Model for Plant Uptake at an Industrial Contaminated Site with a High Variable Arsenic Concentration ,Environments , Vol. 4 (4),pp 67 , 2017, https://doi.org/https://doi.org/10.3390/environments4040067
, Nature-Based Solutions for Restoring an Agricultural Area Contaminated by an Oil Spill ,Plants , Vol. 11 (17),pp 2250 , 2022, https://doi.org/https://doi.org/10.3390/plants11172250
, CO2 footprint analysis of consolidated and innovative technologies in remediation activities ,J Clean Prod , Vol. 297 ,pp 126723 , 2021, https://doi.org/https://doi.org/10.1016/j.jclepro.2021.126723
, Dealing with complex contamination: A novel approach with a combined bio-phytoremediation strategy and effective analytical techniques ,J Environ Manage , Vol. 288 ,pp 112381 , 2021, https://doi.org/https://doi.org/10.1016/j.jenvman.2021.112381
, New Light on Phytoremediation: The Use of Luminescent Solar Concentrators ,Applied Sciences , Vol. 11 (4),pp 1923 , 2021, https://doi.org/https://doi.org/10.3390/app11041923
, Green Roofs’ End of Life: A Literature Review ,Energies (Basel) , Vol. 16 (2),pp 596 , 2023, https://doi.org/https://doi.org/10.3390/en16020596
, Environmental assessment of a new building envelope material derived from urban agriculture wastes: the case of the tomato plants stems ,Int J Life Cycle Assess , Vol. 28 (7),pp 813–827 , 2023, https://doi.org/https://doi.org/10.1007/s11367-023-02152-2
, A simplified method for the indirect evaluation of the 'embodied pollution' of natural stones (marble) working chain to be applied for achieving the Ecolabel brand of the product ,J Clean Prod , Vol. 362 ,pp 132576 , 2022, https://doi.org/https://doi.org/10.1016/j.jclepro.2022.132576
, The use of halophytic plants for salt phytoremediation in constructed wetlands ,Int J Phytoremediation , Vol. 19 (7),pp 643–650 , 2017, https://doi.org/https://doi.org/10.1080/15226514.2016.1278423
, Halophytes as vertical-flow constructed wetland vegetation for domestic wastewater treatment ,Science of The Total Environment , Vol. 583 ,pp 432–439 , 2017, https://doi.org/https://doi.org/10.1016/j.scitotenv.2017.01.090
, - , Hypersalinity: Global Distribution, Causes, and Present and Future Effects on the Biota of Estuaries and Lagoons, in Coasts and Estuaries, Elsevier, 2019
- Food and Agriculture Organization of the United Nations, Food and Agriculture: Key to Achieving the 2030, Agenda for Sustainable DevelopmentRome, Italy, 2016, https://www.fao.org/3/i5499e/i5499e.pdf, [Accessed:19-November-2023]
Halophyte based Mediterranean agriculture in the contexts of food insecurity and global climate change ,Environ Exp Bot , Vol. 191 ,pp 104601 , 2021, https://doi.org/https://doi.org/10.1016/j.envexpbot.2021.104601
, Endophytes and Halophytes to Remediate Industrial Wastewater and Saline Soils: Perspectives from Qatar ,Plants , Vol. 11 (11),pp 1497 , 2022, https://doi.org/https://doi.org/10.3390/plants11111497
, Review of technologies for oil and gas produced water treatment ,J Hazard Mater , Vol. 170 (2–3),pp 530–551 , 2009, https://doi.org/https://doi.org/10.1016/j.jhazmat.2009.05.044
, Prospects in the bioremediation of petroleum hydrocarbon contaminants from hypersaline environments: A review ,Environmental Science and Pollution Research , Vol. 29 (24),pp 35615–35642 , 2022, https://doi.org/https://doi.org/10.1007/s11356-022-19299-4
, Wild Halophytes: Tools for Understanding Salt Tolerance Mechanisms of Plants and for Adapting Agriculture to Climate Change ,Plants , Vol. 12 (2),pp 221 , 2023, https://doi.org/https://doi.org/10.3390/plants12020221
, Physiological, Anatomical and Metabolic Implications of Salt Tolerance in the Halophyte Salvadora persica under Hydroponic Culture Condition ,Front Plant Sci , Vol. 7 , 2016, https://doi.org/https://doi.org/10.3389/fpls.2016.00351
, Effects of salinity on the treatment of synthetic petroleum-industry wastewater in pilot vertical flow constructed wetlands under simulated hot arid climatic conditions ,Environmental Science and Pollution Research , Vol. 28 (2),pp 2172–2181 , 2021, https://doi.org/https://doi.org/10.1007/s11356-020-10584-8
, Salinity tolerance in halophytes* ,New Phytologist , Vol. 179 (4),pp 945–963 , 2008, https://doi.org/https://doi.org/10.1111/j.1469-8137.2008.02531.x
, Drought and Salinity Stress Responses and Microbe-Induced Tolerance in Plants ,Front Plant Sci , Vol. 11 , 2020, https://doi.org/https://doi.org/10.3389/fpls.2020.591911
, Response to Hypersalinity of Four Halophytes Growing in Hydroponic Floating Systems: Prospects in the Phytomanagement of High Saline Wastewaters and Extreme Environments ,Plants , Vol. 12 (9),pp 1737 , 2023, https://doi.org/https://doi.org/10.3390/plants12091737
, - Method for the determination of volatile petroleum hydrocarbons (vph) by gas chromatography/photoionisation detector/flame ionisation detector madep vph, https://www.mass.gov/doc/method-for-the-determination-of-volatile-petroleum-hydrocarbons-vph-by-gcms-0/download, [Accessed:19-November-2023]
- Method for the determination of extractable petroleum hydrocarbons (eph), 2019, https://www.mass.gov/doc/massdep-eph-method-0/download, [Accessed:19-November-2023]
- Method 3510C (SW-846): Separatory Funnel Liquid-Liquid ExtractionWashington, DC, USA, 1996, https://www.epa.gov/sites/default/files/2015-12/documents/3510c.pdf, [Accessed:19-November-2023]
- Method 8270E (SW-846): Semivolatile Organic Compounds by Gas Chromatography/Mass Spectrometry (GC/MS)Washington, DC, USA, 2018, https://www.epa.gov/sites/production/files/201704/documents/method_8260d_update_vi_final_03-13-2017_0.pdf, [Accessed:19-November-2023]